Highly pathogenic avian influenza in Great Britain: evaluation and future actions
Published 30 March 2023
Applies to England, Scotland and Wales
Preface by Defra’s Chief Scientific Adviser
Highly pathogenic avian influenza (HPAI) is a regular occurrence in the UK, but we are in the midst of the largest outbreak ever seen in this country, and an unusually severe global pandemic across bird populations and beyond. A record number of cases of avian influenza virus infections were confirmed through the summer months in 2022 in the UK, and incidents have been at their highest level ever during the winter of 2022 to 2023.
Defra’s Animal and Plant Health Agency (APHA) do an excellent job of leading the government’s response to HPAI outbreaks. Their highly skilled scientific staff are at the heart of the response to HPAI this year, as during outbreaks in many past years. With the unusual scale of the present outbreak, however, and with concern about the range of wild species being impacted by the virus I was keen to bring additional insight to the subject using expert external advisors. Defra’s Science Advisory Council’s Exotic and Emerging Animal Diseases subgroup (SAC-ED) exists to provide input into unusual or emerging animal health risks. Guided by advice from relevant experts, and by input from the Chief Veterinary Office, Christine Middlemiss, I asked SAC-ED, augmented by additional external experts as needed, to advise on four specific issues:
- the host range of the current virus and the potential roles of non-avian hosts
- the possibility of interventions to reduce the impact of HPAI on wild birds
- the potential to supplement current approaches to control with vaccination
- the potential to model the expected future trajectory of the outbreak
The resulting report from SAC-ED, published here, provides highly valued insight which augments the expertise in APHA, and a set of eleven clear recommendations. These insights will help guide Government thinking and actions, and I thank all those who contributed to the report for sharing their expertise and for their work to better understand the present concerning HPAI outbreak.
Professor Gideon Henderson
Foreword
This report documents the findings of an independent expert Scientific Advisory Group in highly pathogenic avian influenza (HPAIG), working within Defra’s Science Advisory Council’s Exotic and Emerging Animal Diseases subgroup (SAC-ED). The group was commissioned by the Chief Scientific Adviser (CSA) of the Department for Environment, Food and Rural Affairs (Defra), Professor Gideon Henderson in consultation with the Chief Veterinary Officer (CVO), Dr Christine Middlemiss.
The HPAIG consisted of independent experts covering expertise in veterinary science, epidemiology and modelling, ecology, virology, ornithology, immunology and social science. Meetings were also attended by experts from Defra, with observers as well from the devolved nations, the Animal and Plant Health Agency (APHA), Food Standards Agency (FSA), and United Kingdom Health Security Agency (UKHSA), to provide input about data and the ongoing situation.
The HPAIG met together on three occasions in December 2022; smaller subgroups also met to address particular aspects of the highly pathogenic avian influenza (HPAI) epidemic on several occasions between meetings. The HPAIG was tasked with addressing four key issues with regards to the current epidemic of HPAI in Great Britain (England, Scotland and Wales):
- the host range of the current virus and their potential roles
- the possibility of interventions to reduce impact on wild birds
- the potential to supplement current approaches to control with vaccination
- the potential to model the expected future trajectory of the outbreak
While Northern Ireland is also challenged by HPAI, it was not considered directly here. The remit of the HPAIG did not extend to consideration of the human health implications arising from HPAI. While no recommendations are made regarding human health, where appropriate, zoonotic risks are noted.
This report represents the consensus views of the HPAIG.
Membership of HPAIG
- Prof. Rowland Kao, Roslin Institute and University of Edinburgh (Chair and Chair of SAC-ED)
- Prof. Piran White, University of York (Deputy Chair and core SAC-ED member)
- Prof. Wendy Barclay, Imperial College London (core SAC-ED member)
- Prof. Paul Digard, Roslin Institute and University of Edinburgh (core SAC-ED member)
- Prof. Neil Ferguson, Imperial College London (core SAC-ED member)
- Prof. Nigel Gibbens (ex-CVO, core SAC-ED member)
- Prof. Stephen Hinchliffe, University of Exeter (core SAC-ED member)
- Dr Sarah Burthe, UK Centre for Ecology and Hydrology (expert member)
- Prof. Dan Haydon, University of Glasgow (expert member)
- Prof. Munir Iqbal, The Pirbright Institute (expert member)
- Dr James Pearce-Higgins, British Trust for Ornithology (expert member)
- Dr Kath Webster (ex-APHA Director of Science, expert member)
- Dr Rob Robinson, British Trust for Ornithology (expert member)
- Prof. Michael Tildesley, University of Warwick (expert member)
- Prof. Lonneke Vervelde, Roslin Institute and University of Edinburgh (expert member)
Executive summary
Avian Influenza Virus (AIV) causes influenza in poultry and game birds. It can also infect many wild bird species as well as mammals, and it is a potential source of pandemic influenza in humans. While AIV usually has little impact on poultry populations, “high pathogenicity” forms of avian influenza can have greater impact, and these occasionally arise and persist for varying lengths of time. Since 2014, a novel form of highly pathogenic avian influenza (HPAI) has been spreading with devastating effect across an unusually broad range of species around the world. Since 2021 there has been an expanded geographical distribution of HPAI with unprecedented numbers of poultry infections and a broader distribution of infection in wild bird species, including in areas of Great Britain previously considered to be low risk and, unusually, in 2022 across the summer months.
As the interplay between virus and host is poorly understood for many non-domesticated animals, it is unclear whether these events have arisen from changes in the virus or alterations to external factors such as changes in species ranges and migratory behaviour, and exposure along migration flyways. Wider environmental stressors that impact on food availability and immunocompetence may also play a role, as may prolonged virus survival in the environment due to changes in the virus, or changes in environmental conditions.
Specific biological factors related to the ability of virus to enter cells and then replicate in them have been identified for many known hosts. Though only partially predictive of susceptibility, this could be used to screen for potential host species. Identifying these species would be especially valuable for rodents and passerines (perching birds) as they could play a role in transporting virus into poultry sheds. There is no evidence yet that mammals play a substantial role in virus circulation, as most affected species (mustelids, foxes, and various marine mammals) are likely to have been exposed mostly via scavenging. However, reports of possible transmissions in sea lion populations and the clearer evidence of sustained circulation in farmed mink population are indicative of the potential for outbreaks in mammals. The circulation in mink is of particular concern, as mink are closely related to ferrets, which are commonly used as experimental models in transmission and cross-species adaptation studies of human influenza.
HPAI may also impact on the conservation of wild birds. In Great Britain, HPAI has been detected in more than 60 wild bird species, including 23 seabird species, many of which are not previously known to have been affected. Reported seabird mortality has been in the tens of thousands. Large mortalities of northern gannets and great skuas are of particular concern due to the global importance of the British populations. Unfortunately, relatively few options are available to manage the impacts of HPAI on wild bird populations and there is uncertainty around the effectiveness of all these options. Carcass removal offers potential for short-term benefits in certain localised situations. Consideration should also be given to extending restrictions on gamebird and waterfowl releases beyond the current regulations, which already prohibit release in areas around outbreaks of HPAI. This would minimise risks of maintaining infection or amplifying infection to other species. Vaccination of wild birds currently poses significant technical and logistical challenges but should remain an option for consideration in extreme situations such as extinction risk. Because HPAI acts alongside multiple other stressors to wild bird populations, it is important to take a precautionary approach regarding any anthropogenic factors that may exacerbate risks, especially for gamebirds, waterbirds, seabirds and raptors, whose populations are vulnerable to HPAI. The global nature of the threat from HPAI and the migratory links between different parts of the world mean that it is vital to promote good practice for reducing transmission risks to wild birds globally as well as in Great Britain.
Poultry vaccination could be used to augment existing HPAI control measures as already occurs in some countries with endemic HPAI. Its use and required specification would depend on a combination of the epidemiological situation (for example, is it becoming endemic?) and policy requirements (for example, eradication or reduction in number of outbreaks?). Vaccination is currently under consideration in several countries experiencing similar challenges to Great Britain, including in Europe. While as yet there are no UK-approved vaccines matched to the current HPAI virus, ongoing trials in Italy, the Netherlands, and France are testing candidate vaccines. Imperfect vaccine performance, and the challenges that arise from potentially dealing with multiple virus strains would have to be considered for any implementation. Vaccination may also best be used by targeting specific industry sectors and geographical areas. Any putative vaccine candidate would need to be tested with commercial breeds of poultry bred from vaccinated hens and field-tested in conditions similar to those experienced by commercial poultry. With sufficient preparation, promising vaccine candidates could be field trialled in the 2023 to 2024 winter season, however this would require commencing appropriate studies now, including modelling, the design of experimental studies, field trials, and the development of tests and protocols to ensure that vaccinated populations are free from disease.
Epidemiological models are useful tools for organising data, evaluating hypotheses regarding mechanisms and patterns of spread, providing projections for epidemic trajectories, and comparing the impact of different interventions. Models already exist that, with appropriate data, can capture the spatial and temporal aspects of recorded poultry infections in Great Britain. Where robust estimates of rates of new infections from wildlife are available, they can provide projections of future epidemic trajectories. Existing data on poultry and game bird populations are in the process of being augmented with data on the patterns of contacts between premises, including company links, and the movements of workers and vehicles. However, to be most useful, these data need to be regularly updated and maintained. Information on transmission pathways from wild birds to poultry and between farms and the relative differences in biosecurity risks in different subsectors, as well as the frequency and severity of biosecurity lapses would be invaluable.
Models of HPAI circulation in the wild bird population offer considerably greater challenges, due to the difficulty of obtaining data on wild species, the complexity of their interactions and uncertainty over which species might play a role in maintaining virus circulation. Nevertheless, such models can be valuable tools for the exploration of future scenarios where HPAI has become endemic in locally resident wild birds. Where possible, field and genomic studies to estimate past exposure to AIV and current levels of infection would be invaluable for developing models to project future behaviour.
Our understanding of the drivers of circulation of H5N1(all avian influenza A viruses are named on the basis of the type of two surface proteins, with 16 “hemagglutinin” (H) and 9 neuraminidase (N) numbered subtypes (resulting in H5N1, H9N2 and so on) in birds), the long-term epidemic trajectory of both the disease and the virus in Great Britain, and its likely longer-term impact on both the poultry industry and on wild birds has many gaps. Thus underpinning recommendations for managing highly pathogenic avian influenza virus (HPAIV) is the need for enhanced surveillance, including:
- expanding the passive surveillance capacity for wild birds
- active surveillance and intense sampling of wild birds in areas around infected premises (IPs)
- enhanced sequencing, preferably from as many virus samples as possible from both wild birds and poultry
Such enhanced capacity needs to be supported by the adoption of rapid serological tests, procedures for inactivating virus in the field to allow safe increases in laboratory capacity and streamlined procedures for training research staff in appropriate procedures. Any solution to the issues of laboratory and surveillance capacity should not only address the requirements for the current HPAI situation, but also be formulated with the expectation that exotic and emergent disease problems are likely to become more frequent and more intense in the future.
1 Introduction
Avian influenza is a disease of poultry caused by infection with avian influenza A viruses (there are four known types of Influenza virus, A, B, C, and D. Only Influenza A is known to infect poultry. It is also the only known source of human pandemics) or “AIVs” (Swayne and Spackman 2013). Avian influenza A viruses affect many species of birds beyond poultry as well as many mammalian species. It is also the source of human influenza, including the likely source for the 1918 pandemic virus (Worobey and others 2014). Wild birds are important for sustaining the circulation of AIV and as a source of poultry infections. All AIVs are named on the basis of the type of two surface proteins, with 16 “hemagglutinin” (H) and 9 neuraminidase (N) numbered subtypes (resulting in H5N1, H9N2 and so on) in birds. In addition, AIVs are further classified into two broad pathotypes, low pathogenicity avian influenza (LPAI) and a high pathogenicity form (HPAI). The designation is based solely on the capacity to produce disease and cause deaths in chickens (Gallus gallus domesticus). All naturally occurring HPAI viruses observed thus far have been H5 or H7 subtypes. Novel HPAI forms arise from either reassortment of existing LPAI and HPAI viruses, or evolution from LPAI (Lycett and others 2019). Remarkably, while reassortment events have typically arisen in countries where backyard farms co-exist with a growing intensive production sector, emergence from LPAI has overwhelmingly been observed in countries with intensive commercial production (Dhingra and others 2018).
Since 2014, HPAI viruses with H5 genes grouped within a single H5NX genetic clade (the 2.3.4.4 clade, within the Goose/Guangdong or Gs/Gd lineage) have spread globally, resulting in severe outbreaks across much of the world. Patterns of spread have also been unusual, for example, the trans-Atlantic migration to North America in 2021, and recent spread to Central and South America (Lee and others 2017). These viruses have been the cause of devastating HPAI outbreaks in poultry and they pose a threat to food security in many parts of the world. Between October 2021 and September 2022, one particular H5N1 subgrouping (Clade 2.3.4.4b H5N1) has been the predominant cause of a total of 2,520 HPAI outbreaks in poultry, 227 outbreaks in captive birds, and 3,867 HPAI virus detections in wild birds in Europe. (it is this lineage of the HPAI virus and its epidemiology that will be referred to throughout this report, unless otherwise specified). Across the 37 countries affected, over 50 million birds have been culled (EFSA 2023).
The observed host range of this clade has been both unusual and resulted in severe impacts. In Great Britain and in other places worldwide, mass die-offs of seabirds not previously known to be affected have occurred. Poultry have also been infected in many areas of Great Britain previously considered to be at low risk. This is probably due to an altered geographical distribution of HPAI virus infection in wild birds (see Figure 1). Unusually, outbreaks on poultry farms have persisted across the summer months and considerable numbers of non-migratory Great Britain birds have been found to be infected. This has led to concerns that these unusual patterns of HPAI virus circulation could persist. The scale of the incursion into Great Britain during the winter seasons of 2020 and 2021, and 2021 and 2022 and the unusual, continued outbreaks during the summers of 2021 and 2022 have presented a substantial case for an evaluation of the evidence surrounding the current epidemiology and impact of HPAI in Great Britain.
Future actions to monitor and control HPAI in Great Britain will depend on several interlinked factors – first, the trajectory of HPAI risk due to importations of virus with migrating wild birds, second, the ability of the virus to persist in domestic wild populations (including both non-migratory birds and potentially mammals), and the extent to which spread from kept-bird premises, including farms, game bird and zoological collections, is likely to occur. It must also consider the direct impact on the wild bird populations, especially where there is a conservation risk and where infections in wildlife may put kept birds at risk. Thus in response to the current situation, the Defra Chief Scientific Adviser convened a task and finish group of scientific experts to address four key questions:
- What is the potential role for the involvement of other animal reservoirs?
- Is there any more we can do about wild birds?
- Is vaccination a viable route forward?
- What is the expected future trajectory of the outbreak (this 2023 and beyond)?
For each question, the report will discuss the relevant scientific evidence on the subject, identify important data gaps and areas requiring better understanding, make recommendations for further data collection and, where appropriate, identify timely measures that could be effective in mitigating the current epidemic spread. It will highlight the key recommendations that are deemed most important based on:
- the robustness of their scientific evidence
- the ease of implementation
- the potential impact on the future of HPAI in Great Britain
Finally, while the risk of zoonotic spread and evolution of greater zoonotic potential will always be a concern, this is a question that is largely distinct from the Terms of Reference of this report. (See also Avian influenza (influenza A H5N1): technical briefings).
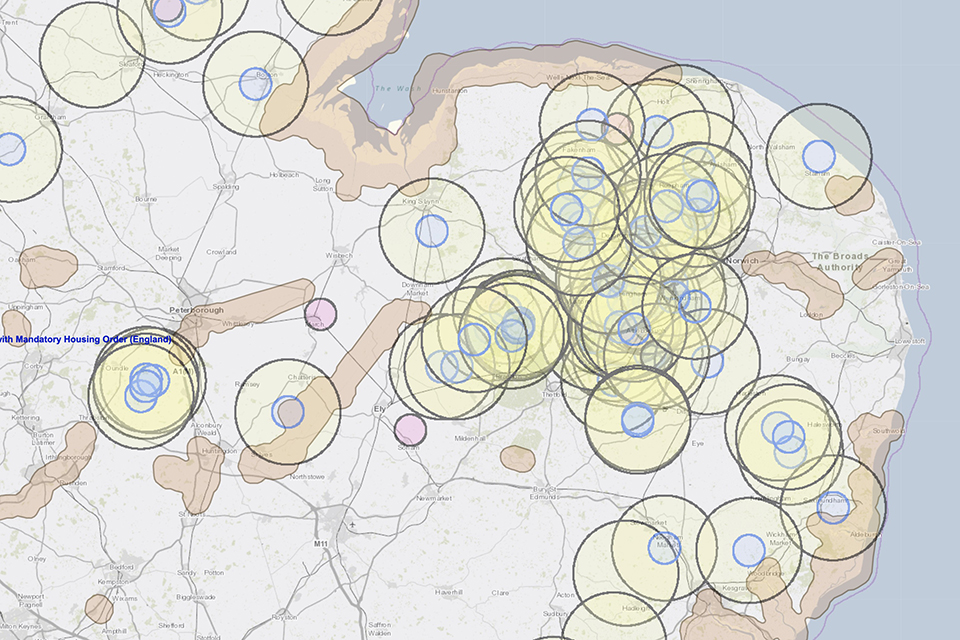
Figure 1: Map showing East Anglia cluster 3km HPAI Protection Zones (higher risk controls, shown as blue circles around confirmed HPAI cases in poultry), 10km HPAI surveillance zones (yellow circles), and 3km Captive Bird monitoring Controlled Zones (lower risk controls around confirmed HPAI cases, shown as pink circles).
The map in Figure 1 shows three types of disease control zones. Three km radius protection zones are placed around confirmed cases of HPAI in poultry Protection Zones (higher risk controls, shown as blue circles around confirmed HPAI cases in poultry), 10km radius surveillance zones surround the protection zones (yellow circles), and around confirmed cases of HPAI in captive birds, there is placed a 3km captive bird monitoring controlled zone (lower risk controls around confirmed HPAI cases, shown as pink circles). In these zones, there is expected to be an enhanced risk of HPAI outbreaks in kept birds. Also shown on the map are areas which are designated as being likely at high risk of infection, largely due to their location along migratory bird flyways and therefore risk from HPAI infected migratory birds (shaded areas). Comparing the locations of the control zones to the traditional high-risk areas, there is an obvious substantial difference. On this map, this is particularly marked in an area around East Anglia. This East Anglian concentration of control zones is both outside the traditional high-risk areas, and also well away from the seashore (where most of the high-risk areas are concentrated. The area shows both many outbreaks of HPAI in poultry and substantial overlaps amongst many protection and surveillance zones.
2 Host range and reservoir potential of H5N1 HPAI virus
2.1 Background
While the recent pattern of outbreaks in Great Britain could have been caused by chance, the persistent and global nature of the problem make it more likely that the recent epidemiological patterns observed in Great Britain could be explained by at least one of, or a combination of virus-related drivers including:
- the ability to infect a greater range of potential wild bird species which are not normally susceptible, thus broadening the sources of infection for poultry and providing the means to persist in Great Britain over the summer
- increased ability to infect its existing range of hosts, thus resulting in more frequent presentation of infection, and therefore greater opportunities for spillover infection.
- greater shedding of virus from some host species following infection
- longer survival in the environment, promoting greater exposure for the same level of shedding from each bird, and again resulting in a greater observed host range
Circulating HPAI virus in Great Britain and elsewhere has undergone several reassortments (meaning mixing of genetic material with other AI viruses) and therefore, there is considerable potential for virus characteristics to have substantially changed. However, we do not yet know if this has resulted in a substantial selection advantage, and, while experimental studies may identify these under controlled conditions, such changes would be difficult to quantify in the field. Thus, the current epidemiological observations could also result from environmental or ecological changes that could allow the virus to establish itself in populations not normally exposed or made more susceptible (for example, due to environmental stress), which then provides the enhanced infection pressure and results in over-summering. This is discussed further in Section 3 below. None of these hypotheses are inherently exclusive and some aspects are either untestable or unfeasible. However, some investigations are possible that would narrow this range of hypotheses, thereby providing valuable insights to inform risk assessments and future projections of epidemic trajectory.
Whatever the reasons behind it, the scale and breadth of the current outbreak provides new opportunities for the virus to infect a variety of Great Britain animal species, both avian and mammalian. In turn, this would create opportunities for virus evolution, potentially establish new reservoir hosts, further hindering control efforts and increasing the impact of the epidemic. For example, newly infected species could pose risks for introducing virus into poultry sheds (for example, passerines or perching birds or rats as vectors). They may also enhance opportunities for additional spillover events (for example, into humans or domesticated species), and therefore provide new opportunities to drive viral adaptation in unwanted directions (for example, further adaptation to mammalian species).
2.2 Host range
The primary global animal reservoir for influenza A viruses is widely accepted to be wild birds from the orders of Anseriformes (ducks, geese, and swans) and Charadriiformes (gulls, skuas and wading birds), but particularly various species of dabbling ducks (Yoon, Webby and others 2014). Other species including mammals can also act as reservoirs for specific lineages of the influenza A virus, but as a rule, most AIVs do not result in onward transmission from infected mammals unless they first accumulate adaptive mutations. While such a rule cannot be taken as absolute, the susceptibility to disease of individual species for any single strain of virus ranges from very high (for example, chickens, turkeys) through tolerant (for example, mallard ducks, geese and crows, that support virus replication without severe disease) through to almost entirely resistant (for example, pigeons, that can be refractory to infection).
The factors influencing influenza virus host range are multiple, but two facets are accepted as playing major roles. The first facet is if the receptors which the virus uses to enter host cells are well matched to binding by the viral haemagglutinin (HA, the protein responsible for binding to the target cells). Viral haemagglutinin has an identifiable preference for receptors comprised of different forms of a carbohydrate called sialic acid. These receptors are found on the epithelial cells that the virus targets. Differences in the types and distribution of sialic acid are seen as the primary difference determining infection of avian and mammalian species. However, more subtle modifications to sialic acid characteristics (for example, molecular chain length, branching pattern, sulfation and other modifications) also affect recognition by HA and thus the ability for viruses to affect different cell types (Zhao and Pu 2022).
The second facet is if the viral polymerase (an enzyme that drives virus replication) can work with the host “ANP32” proteins to enable replication (Long and others 2019). Differences in ANP32 are found between mammals and birds (Matilla and Radrizzani 2005). When comparing expression in chickens and humans, ANP32 has been shown to play an important role in setting AIV host range (Long and others 2019). However, virus replication relies on multiple other interactions with other host factors, many of which also affect host range, even in the absence of prior immunity (Long and others 2019).
This interplay between virus and host is reasonably well understood and partially predictive for some species (for example, humans, chickens, pigs) but much less so for many others, especially non-domesticated animals. Knowledge of sialic acid distribution in wild birds does not cover the current diversity of afflicted bird species and is biased towards North American and Eurasian species (Costa and others 2012, Franca and others 2013, Verhagen and others 2021). Similarly, genome sequence information that would inform us about ANP32 expression is lacking for many avian species of current interest, despite progress on the “B10K” project to sequence all bird genomes.
Determining the precise receptor specificity of the current H5 HAs would also be useful. Some work is in progress under the UK Research and Innovation (UKRI) funded Flu-Map project that is being conducted between researchers at the Pirbright Institute and Imperial College London (ICL) (this is a multi-institutional research project led by the APHA and funded by UKRI and Defra). Testing polymerase usage of ANP32 proteins from a wider variety of species is also underway at ICL under Flu-MAP but requires further information from those with access to materials and, or genome sequences from wild bird species (potentially via FluMap consortium partners APHA and the Roslin Institute and, or University of Edinburgh and via Nature Scot). Comparative studies with previous H5 viruses would also enable some characterisation of potential host range differences, providing evidence to examine the genetic change hypotheses listed above.
2.3 Potential spillover or reservoir hosts
The increased prevalence of the current H5N1 virus strains and the variety of bird species being infected with them gives further opportunities for the virus to expand its host range. Where there is no adaptation of the virus, “spillover” infections may have severe consequences for the infected host, but with limited risk of onward transmission, and minimal consequences for populations as a whole unless the conditions for continued spillovers persist for a long time and over a substantial area. However, the known ability of the virus to rapidly adapt to a new host and establish productive infection cycles (with viral replication, even if it does not result in severe disease) means that every spillover event increases the opportunity for new viral reservoirs to become established in Great Britain. The establishment of these new reservoirs, in turn has the potential to expose other species, potentially further expanding the host range. Such novel reservoirs might come from animal species not previously known to function as such, but could also represent specific Great Britain populations of known reservoirs (such as duck species) that do not normally maintain the virus here.
Key knowledge gaps for avian reservoirs are H5 incidence in Great Britain resident duck species and passerines. When considering resident duck populations, mallards are a likely host, given that they play this role elsewhere in Eurasia (Yoon and others 2014). Passerine species should also be considered, given their prevalence in Great Britain and potential role as a bridging species between migratory wild birds and poultry (Shriner and Root 2020, Le Gall-Ladeveze and others 2022). For instance, house sparrows are susceptible to laboratory H5N1 infection and tree sparrows can transmit the infection to co-housed chickens (Brown and others 2009, Yamamoto and others 2013).
When considering the risks of further spillover events into Great Britain mammals, current and historical epidemiology as well as experimental tests of Gs/Gd lineage H5 HPAIVs in non-avian species, together with knowledge of the molecular-genetic determinants of influenza virus host range, allows a tentative classification of specific species into relative risk categories (see Table 1).
Table 1. Summary of potential risks of infection with H5NX HPAIVs across mammalian species
Risk of becoming infected | Relevant species | References |
---|---|---|
Very Low | Ruminant Species | (Sreenivasan and others 2019) |
Low | Horses, Humans | (Abdel-Moneim and others 2010, Hamed and others 2014) |
Low-to-moderate | Cats, dogs and pigs | (Kuiken and others 2004, Songserm and others 2006, Maas and others 2007, Ma and others 2008, Lee and others 2018) |
High | Mustelids, foxes and seals | (Floyd And others 2021, Rijks and others 2021) |
Unclear, but potentially important | Lagomorphs, rodents | (Velkers and others 2017, Root and others 2018, Cummings and others 2019) |
Ruminants (cows, sheep, goats, deer) are classified as very low on the grounds that influenza A virus isolates from these species are sporadic, rare and tend to be of human origin rather than avian strains (Sreenivasan and others 2019). Equids are judged to be low risk because infection events with Gs/Gd lineage H5 HPAIVs seem to be at best rare, though there is some evidence in donkeys (Abdel-Moneim and others 2010, Hamed and others 2014). In humans, virus receptor tropism and ANP32A usage coupled with epidemiological observations suggest a (currently) low rating. Pigs are viewed as a gateway species for cross species transmission of influenza viruses, based on their frequent proximity to farmed poultry and humans and their intermediate presentation between these two forms of sialic acid distribution and ANP32A function in viral replication (Ma and others 2008). Nonetheless while there is some evidence for their natural infection with H5 HPAIVs (Chauhan and Gordon 2022), experimental tests of more recent H5 strains in pigs do not indicate high susceptibility to the virus (for example Kaplan and others 2017). Cats, both companion animal and zoo and wild species, are known to be susceptible to infection with H5 HPAIV, including both from contact with infected poultry and via experimentally after eating infected birds (Kuiken and others 2004, Lee and others 2018). As of December 2022, there is at least one example of natural infection with H5N1 (see World Health Organisation for animal health report of infection in a cat). Similar reports of infection following scavenging behaviour in domestic dogs also exist (Songserm and others 2006), although experimental challenge studies are more equivocal (Maas and others 2007). Animals placed in the high-risk category are those known to have been infected in substantial numbers during the current outbreak. These include land animals: foxes and various mustelids (otters, badgers and mink), bears, skunks, raccoons and opossums, as well as marine species (seals, dolphins and porpoises). While these instances are presumed to result from scavenging behaviour (Floyd and others 2021, Rijks and others 2021; see also United States of America HPAI detections for 2022 to 2023), a report of mass mortality of infected sea lions in Peru is one example where viral circulation within a mammalian population cannot be ruled out, though it also may be due to mass exposure to infected seabird carcasses. Evidence of circulation amongst mammals is more definitive for an outbreak amongst mink under farmed conditions (Agüero and others 2023). Virus isolated from this outbreak display several mutations that include some reminiscent of human influenza A viruses (Aguero and others 2023, de Vries and de Haan 2023). However, similar mutations have also been previously observed in H5N1 viruses without evidence of further substantial adaptation to mammals. Nonetheless transmision in mink is of particular concern, given that the closely related ferret is regarded as a good model for the behaviour of influenza viruses in humans (Belser and others 2018).
A final category of “unclear” is also considered. This category includes common Great Britain mammal species in the rodent family (for example rats and mice) where epidemiological and experimental data are lacking. Experimental challenge data are mostly from laboratory species of rodent and thus of questionable relevance to wild Great Britain animals. Surveillance of AIV in wild rodents has generally given negative or low-level positivity of virus (by serology) in rural areas (Velkers and others 2017), although one survey of an urban area using polymerase chain reaction (PCR) tests (which amplify identifiable genetic fragments to indicate presence of virus), returned startlingly high levels of apparently virus-positive samples that could not be sequenced or subtyped (Cummings and others 2019). Nevertheless, the numbers of rodents and their association with farms makes them potentially significant spill over and, or vector species (Velkers and others 2017). Data for rabbits, another common Great Britain wildlife species, are also in short supply. Cottontail rabbits can be infected with Clade 2.3.4.4 H5 HPAIV and will shed virus (Root and others 2018) but it is unclear if this extrapolates to the European rabbit.
The categorisations listed in Table 1 are indicative judgments only. Influenza A virus is highly mutable and exists in a constant state of evolutionary flux. Furthermore, specific viral strains can violate the perceived “rules” that set host range and, or disease severity if infected (for example, the 2009 H1N1 influenza pandemic would not have been predicted to replicate well in humans by the then current state of understanding (Mehle and Doudna 2009)). Thus, the two factors of sialic acid species and ANP32 protein family, respectively indicate receptor suitability and potential for replication should be viewed as, at best, a broad indicator of potential host competency to becoming infected. It does not provide definitive insight into the ability to infect other hosts.
Nevertheless, in a resource limited setting, the determination of sialic acid species and distribution and ANP32 protein family gene sequences and function are an important step in establishing the potential host range and may provide a guide for prioritising surveillance efforts designed to detect spillover events. Further consideration should also be given to the likelihood and consequence of a particular host species becoming a reservoir in the event of the infection becoming established. For example, it is reasonable to postulate that mallard ducks would have a higher probability of reaching reservoir status in Great Britain given their role elsewhere in the world, and with perhaps higher consequences for further circulation than lower density mammalian predators for instance.
3 Feasibility and options for controlling transmission in wild birds
3.1 Background: How and why is this HPAI outbreak different to previous outbreaks
The 2021 and 2022 avian influenza season, which started in October 2021, has had unprecedented impacts on wild birds in terms of the magnitude and the range of species affected, as well as the rapidity and routes of spread both nationally and internationally. It has had extensive impacts across 37 European countries with 710 wild bird detections from 11 June to 9 September 2022 and mass mortalities in colonial nesting seabirds in France, Netherlands, Germany and the UK (EFSA 2022). In the UK, more than 60 species had positive detections for HPAI associated with mortality events during the 2021 and 2022 season (by end September 2022). This included 23 seabird species, the majority of which had not previously been reported as being infected with HPAI.
Although there is a suite of wild bird population surveillance schemes coordinated by the British Trust for Ornithology (BTO) through a long-term partnership with the Joint Nature Conservation Committee (JNCC), Royal Society for Protection of Birds (RSPB) and others that provide long-term monitoring data, we do not yet have a full assessment of the level of mortality and population-level consequences of the 2021 and 2022 avian influenza season on wild birds. Submissions of data for 2022 are still being entered, and many seabird colonies were not surveyed in 2022, due to access restrictions because of HPAI. Moreover, the complex population structure and longevity of many seabirds means that population-level impacts at individual colonies may not become apparent for several years.
Mortality data collated by individual country nature conservation bodies (CNCB) and the RSPB during the 2022 breeding season provide a partial assessment of impact. These data suggest unusually high levels of mortality, both in host species where HPAI has not been previously recorded, but also in species such as gulls in which HPAI is known to have circulated in the past. More than 8,000 individual northern gannets, 4,000 common guillemots, and 2,700 great skuas were reported dead, likely due to HPAI, along with more than 1,000 individual Sandwich terns, black-headed gulls, black-legged kittiwakes and herring gulls. These values compare to an estimated UK population of 293,200 apparently occupied gannet nests (AON) and 9,600 apparently occupied great skua territories (AOT), both of which represent more than 50% of the world population. This makes large, reported mortalities of both species of global significance. Mortalities for Sandwich tern compare against a UK population of 12,500 AON; and for the gulls,138,000 black-headed gull AON, 139,200 herring gull AON and 378,000 kittiwake AON (JNCC 2021), whilst an estimated minimum 30% of the small UK breeding population of roseate terns was lost from the only Great Britain colony on Coquet Island.
Analyses of ringing recoveries (reports of dead ringed birds to BTO) suggest that the numbers of reported recoveries in 2022 were unusually high for great skua (Figure 2), northern gannet, common guillemot, Sandwich tern, common tern, Arctic tern and black-legged kittiwake. Overall, these figures on seabird mortality will be minimum estimates, with many mortalities likely to have been undetected.
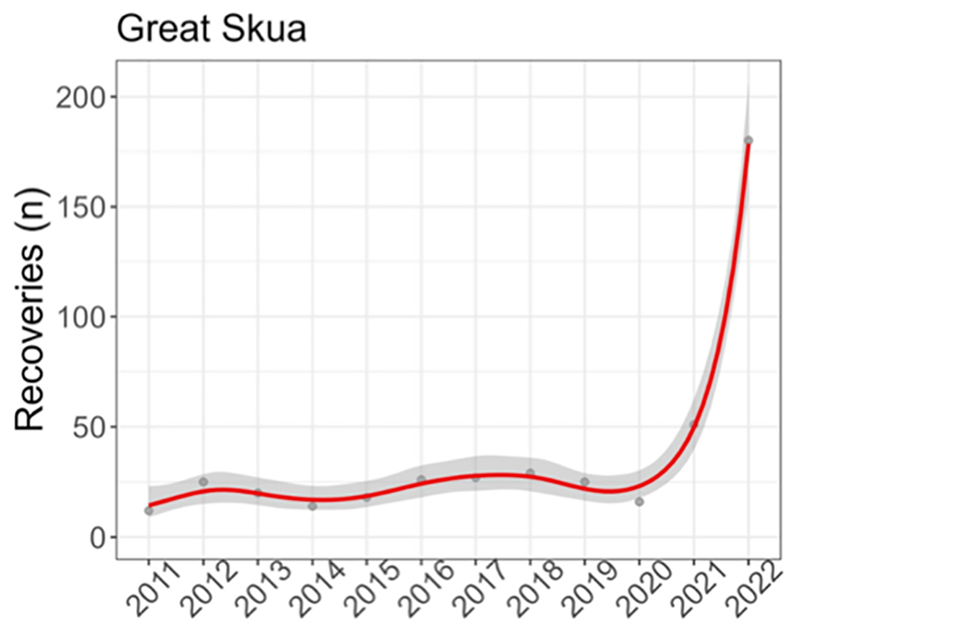
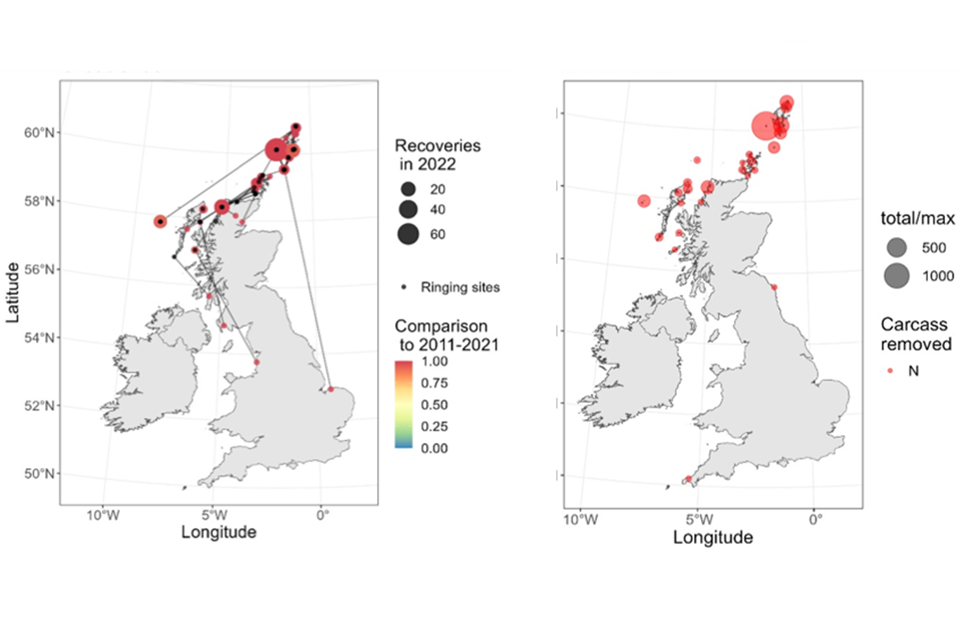
Figure 2: Changes in the number of dead great skuas (recoveries) per year reported to the BTO through the BTO and JNCC ringing scheme (top) to the end September 2022.
Figure 2. shows the changes in the number of dead great skuas (recoveries) per year reported to the BTO through the BTO and JNCC ringing scheme (top) to the end September 2022. This is presented in three panels. In the first panel, changes in the number of dead great skua recoveries of ringed birds per year reported to the British Trust for Ornithology or BTO through the BTO and JNCC ringing scheme (top) to the end September 2022. The red line with grey estimates of uncertainty indicates underlying changes through time, with the numbers for each year given as grey circles. From 2011 to 2020, the number of reported dead skuas shows some slight variation but is reasonably steady and predictable and always that less than 50 per year, and more typically approximately 25 per year. However, in 2021 the figure reaches 50 for the first time due to the smaller but still substantial HPAI outbreak in that year, as described in the paper by Banyard and others published in 2022. In 2022, the figure jumps substantially to 175. The spatial distribution of the 2022 recoveries (bottom left) is compared to the number of mortalities reported to Country Nature Conservation Bodies (CNCBs) (bottom right). On the map of recoveries, the lines join the location of dead birds reported away from colonies to the colony they were ringed at, and the colour indicates the proportion of mortalities reported from 2011 onwards that were in 2022. In both cases this is presented across a number of sites in Great Britain. The maximum number of approximately 60 recovered ringed birds occurs at one location in the Shetlands, but with substantial numbers all along the coastline of Scotland, and a few recoveries along both the east and west coasts of England. In several cases, including all the recoveries in England, the ringing sites are farther north than the location of recovery, and all of these are in Scotland. The reported number of recoveries at each site is coloured to indicate the proportion of mortalities reported from 2011 onwards that were in 2022 – in all cases, this proportion was close to 100%. A direct comparison between the two panels shows that there is a strong correspondence in the distribution of recovered ringed birds to the distribution of the number of carcasses, with sites having higher numbers of recovered, ringed birds, also having higher numbers of carcasses. The highest number of carcasses (about 1000) is found at the same site with the highest number of recoveries. This figure is taken from a report for BTO by Pierce-Higgins and others, published in 2023.
The 2022 outbreak was unusual not just in Great Britain, but globally. Detections of HPAI in seabirds in Europe from June to September 2022 were 49 times greater than in the previous year, substantially affecting not just Great Britain but also France, the Netherlands and Germany (EFSA 2022). Although there has been previous intercontinental spread of HPAI, including to North America (Ramey and others 2022), the first 2021 to 2022 detection in North America of the Eurasian strain of HPAI H5N1 occurred in December 2021 in Newfoundland and Labrador, Canada, and appeared to be spread by transatlantic migratory birds (Caliendo and others 2022). This appears to have spread across North America where it has devastated colonies of a range of species including northern gannets, puffins and common murres (guillemots) in a comparable way to Great Britain. The virus now appears to have spread to several South American countries where it is also causing significant mortalities in wild birds, poultry and sea mammals, as well as one case of human infection. Outbreaks have also been apparent in South Africa across a range of species, notably in 2018 and then 2021, and may again be returning this austral breeding season.
3.2 Drivers of the current situation in wild birds
There are several factors which may have contributed directly or indirectly to the 2021 and 2022 HPAI observations in wild birds. Although the virus is changing continuously, there is no indication that any specific changes in the virus have been associated with the increase in HPAI in 2021 and 2022 (see also Section 2 above). We therefore consider other potential drivers below.
3.2.1 Changes in abundance of the virus and the potential for novel spill-over into breeding populations
It is likely that many seabird populations were always vulnerable, but that they had not been exposed in previous years. However, starting in October 2021, there was a greater overall range and abundance of the virus across Great Britain, particularly in wintering waterbirds which are more typically associated with lakes and rivers, as exemplified by the large mortalities of Svalbard barnacle geese on the Solway Firth (located off the south-west coast of Scotland, between Dumfries and Galloway and England ). This broader range could have increased the likelihood of the virus being transferred to naïve seabird populations. Plausible transmission routes include migrating wildfowl returning to their northern breeding grounds, or gulls that associate with wintering wildfowl but intermix with other seabirds at colonies during the breeding season. Little is known about seabird pelagic movements, and there is insufficient evidence to draw any conclusions regarding specific routes of transmission at present. The greater infection pressure from the virus and the variety of bird species affected also provides new opportunities for the virus to adapt and potentially for further spillovers to occur involving other animal species.
3.2.2 Changes in the range, abundance and migratory or seasonal behaviour of wild bird species
There is evidence that waterbird migration distances have shortened in relation to climate change, and it has also been suggested that long-distance waterbird migrants are migrating earlier and short-distance ones migrating later. The combined effect of these patterns means that there is the potential for increasing overlap between different species in breeding, resting, and feeding grounds, resulting in potentially higher densities of birds and a higher risk of inter-species transmission. However, whether waterbird migratory movements in 2022 were unusual in relation to this longer-term trend is currently unknown.
For seabirds, some Great Britain populations have increased significantly over the last 20 years (for example, gannet by 34%, great cormorant by 16%, black-headed gull by 26%, guillemot by 60%, and razorbill by 37%, great skua by an undefined amount) (JNCC 2021). Increasing populations of certain species, such as gannets, may lead to higher breeding densities, potentially increasing HPAI transmission in those species. In some species, increased population density may also have led to more movements of juveniles prospecting for sites and potentially increased movement of avian flu between colonies.
In contrast to waterbirds, there is little evidence for any changes in distance or timing of migration for seabirds. Changes in the timing of migration may influence interactions on seabird colonies. However, we know very little about seabird movements away from the colony, and changes would be difficult to detect.
3.2.3 Changes in exposure along the flyway
Many of the wild bird species that show high levels of HPAI are migratory species and move large distances on their migrations. Two migration pathways provide potential routes for avian influenza to reach Great Britain in autumn and early winter: the Black Sea-Mediterranean flyway which connects Europe with Africa, and the East Atlantic flyway which links Arctic breeding grounds stretching from eastern Canada across to Siberia with wintering areas in West Africa and western Europe. However, there are six other major flyways across the world and there is some degree of overlap across all major flyways in the Arctic (Galbraith and others 2014). Hence, there is potential for HPAI to be transferred both between species using the same migratory routes and between those species using different routes, especially at critical bottleneck sites which serve as migratory stopovers for many species. Moreover, the close interface between poultry and wild birds in some parts of the world, especially in parts of Asia, provides opportunities for HPAI transfer from domestic birds to wild birds migrating along flyways.
Phylogeographic (which is the use of evolutionary history of viruses sampled from different geographical areas, to recapitulate movement patterns – see also section 5 below on phylodynamic models) research on AIV across birds using the Pacific, Central, Mississippi and Atlantic flyways in North America has confirmed that migratory movements play a key role in virus dispersal (Fourment and others 2017). There are no data that provide direct evidence of the involvement of specific migratory flyways in the emergence or high levels of HPAI in Great Britain in 2021 and 2022. However, the involvement of multiple species and existing evidence on the role of flyways suggests that it is likely that changes in exposure along the flyway have been a contributory factor.
3.2.4 Changes in behaviour and susceptibility of birds resulting from wider environmental stresses.
Migratory bird species face a wide range of threats. Many of these, such as habitat loss and degradation, climate change, extreme weather events, invasive species, hunting and human disturbance, are common to all bird species. However, certain threats pose a particular risk to migratory species, including loss of critical resting sites. For migratory seabirds, reductions in food supply due to climate change (Mitchell and others 2020) and overfishing (Church and others 2018) can contribute to species declines, especially for species that are unable to adjust their diets to changing distributions and abundance of key resources such as sand eels. For some species, such as northern gannet, direct mortality and disturbance from offshore wind farms poses an additional threat (Dierschke and others 2016). Climate change resulting in warmer temperatures can also have direct impacts on species that are sensitive to heat, causing greater heat stress and declines in productivity.
Climate change may also be especially significant for seabirds through its effects in increasing air and sea temperatures and associated ecosystem-level impacts affecting food resources (Pearce-Higgins 2021). Some birds that breed at high latitudes are susceptible to heat stress in milder temperatures. Great skuas bathe in rock pools at their breeding sites to reduce heat stress (Oswald and others 2008). Increasing use of rock pools by multiple individuals in milder temperatures may result in more opportunities for transmission of infections such as HPAI.
The specialised diets of many seabirds make them especially susceptible to any climate-induced changes in food resources. The increasing overlap between different species in breeding, resting and feeding grounds, as a consequence of changes in the timing of migration movements, will also result in greater food stress and potentially social stress. Food stress resulting from over-fishing or changes in fishing practice can also cause some species to modify their feeding behaviour, for example, great skuas may switch from scavenging on fish discard to greater predation of seabirds (Perkins and others 2018), and also scavenge more on carcasses of dead birds, thus potentially increasing their direct exposure to HPAI virus. Other environmental factors such as pollution can increase physiological stress and reduce immuno-competence (King and others 2021). Birds may also avoid or be displaced from foraging habitats by offshore wind farms (Furness and others 2013), potentially affecting foraging and increasing nutritional stress, and hence making them more susceptible to a variety of infections including HPAI. Should infectious diseases more broadly increase because of these generic stressors, co-infections may also become an issue.
3.2.5 Assessment of the likelihood of different drivers of the current levels of HPAI in wild birds
The different potential drivers of the current HPAI situation are unlikely to act on their own. Moreover, the effects of HPAI infection could themselves exacerbate the impacts of these different drivers. Nevertheless, an evaluation of the likely relative contribution of the different drivers can clarify the management options that may be available to reduce the risk to wild birds, as well as in prioritising future research needs. The Assessment of the factors that may be contributing to the current HPAI situation in wild birds therefore summarised an expert judgement of the likely relative contribution of the different potential drivers, using the Intergovernmental Panel of Climate Change’s (IPCC) guidance framework, which allows uncertainties to be expressed in a probabilistic manner (Mastrandrea and others 2010).
Assessment of the factors that may be contributing to the current HPAI situation in wild birds
Likely (66 to 100% probability):
- virus more widespread, including new introductions to remote seabird colonies
- virus more abundant, causing greater overall infection pressure
- changes in host range, abundance and, or migratory behaviour
- changes in exposure along the flyway
About as likely as not (33 to 66% probability):
- virus over-wintering better
- warmer climate and seas resulting in increased heat stress
- food stress resulting in changes in behaviour such as greater scavenging
- changes in the virus (see Section 2.2 on host range)
3.3 Key evidence needs relating to potential drivers of HPAI in wild birds
3.3.1 Changes in abundance of virus, including changes in seasonal patterns
Further information is needed on how HPAI is maintained over the summer period and over the winter. To support this, we need to understand more about which species are susceptible, how species vary in the responses to HPAI including case fatality ratios, and the population or community size needed for the virus to persist. We also need more evidence on sources of infection and routes of transmission involved in the circulation of the virus among wild birds, and in the circulation of the virus between wild birds and poultry.
It is important to know whether HPAI is now circulating in some species of seabirds all year round or being reintroduced by species that are present during the winter. It is known that the virus can persist in the environment for longer periods in the right conditions, for example, in colder temperatures (Martin and others 2018), but at present the epidemiological significance of environmental persistence is not understood.
Whole genome sequencing (WGS) can be used to characterise the entire viral genome. Sufficient WGS samples taken from a circulating viral population maximises the potential of phylogeographic and phylodynamic analyses which can be used to deliver new understanding of host susceptibility and the dynamics of transmission between populations and species, and how these relate to changes in the virus (Kao and others 2014). However, there needs to be an increase in both targeted and opportunistic sampling (sampling live birds as well as carcasses, with consideration also given to environmental sampling), alongside an expansion in the use of our lab testing capabilities, in order for this potential to be fully realised.
3.3.2 Changes in the range, abundance and, or migratory behaviour of wild bird species
For traditional long-distance migratory species, there is some evidence that spring migration is happening earlier. There is also evidence that some autumn migration is happening later. For example, ducks and waders are migrating to Great Britain from eastern Europe less frequently, and instead wintering around the Baltic or elsewhere on continental Europe (Pavon-Jordan and others 2019). However, the responses of individual populations within these overall patterns are much less well known, with populations of many species comprising individuals with a varying propensity to migrate – so-called partial migration. Very little is known about how these dynamics are changing and the extent to which Great Britain is playing host to birds from a changing mix of countries and regions. The potential implications for the transmission of HPAI (both into and within Great Britain) remain unclear.
There is a research need for modelling and data analysis studies (see Section 5 below) to investigate questions such as the extent to which:
- differences in the impacts of HPAI between colonies and aggregation points are likely to be due to species composition (including presence of, for example, non-breeding gulls or wintering wildfowl
- infection density reflects densities of birds or previous exposure
- pinnipeds have the potential to act as reservoir hosts given the evidence that grey seals carry endemic LPAI (Puryear and others 2016), and the recent suggestions of possible circulation amongst Peruvian sea lions (Gamarra-Toledo and others 2023)
- extreme outcomes (such as mass mortality events) are a consequence of purely stochastic events
Information on the range and abundance of wild bird populations is available through programmes such as the Seabird Monitoring Programme (SMP), the Wetland Bird Survey (WeBS), the Goose and Swan Monitoring Programme (GSMP), the Winter Gull Survey (WinGS) and Avian Demographic Schemes (ADS), that provide information on productivity and survival. The need to increase coverage of these schemes to better understand impacts of HPAI and inform future vulnerabilities should be considered, whilst the WinGS survey dates from 2003 and 2004 to 2005 and 2006 and therefore should be repeated to produce updated information on autumn and winter gull abundance (Pearce-Higgins and others 2023). However, more information is needed, especially in relation to dispersal and migration patterns (the latter especially for seabirds) and more formalised recording of mortality. Expanding the coverage and intensity of demographic data at colonies would improve our understanding of seabird demographic processes, make it easier to separate out seasonal differences in mortality due to HPAI, and disentangle the effects of HPAI from other impacts. Enhanced ringing effort directed at wildfowl and other waterbirds, particularly for the internationally important wintering assemblage, would allow us to better track the impacts of HPAI in the context of other pressures along the flyway.
There is a need for more evidence relating to the behaviour of wild birds. Specifically, we need to have a better understanding of how any changes in range, abundance or habitat use may result in greater direct exposure to the virus through increased interactions with other species. Gulls, which are known vectors of pathogens, have been making increased use of urban environments in the last 30 to 40 years during breeding seasons. This may be partly in response to declines in food availability due to closures in landfill sites (Langley and others 2021), declines in nearshore fishing discards, and increased foraging ranges. Analysis of existing tracking data and collection of new data in the context of HPAI risk would help to disentangle these different factors. Ornithological assessments for all IPs are conducted by field staff and these feed into operational decision-making. However, the uncertainty in the current evidence supports the need for more systematic scientific investigations on the interactions of wild birds with poultry farms to test specific hypotheses regarding mechanisms of transmission and inform management options to reduce the farm-level risk of transmission of HPAI from wild birds to poultry.
There is a need to understand how changes induced by human activities or other environmental or climatic changes may cause increased physiological stress, and the extent to which this increased stress may result directly in increased susceptibility through a depressed immune response, or indirectly through its effects on diet and foraging behaviour, such as greater scavenging. This information is likely to lead to a more detailed understanding of the impacts of HPAI on species such as great skuas, and potential implications for other species associated with them.
3.3.3 Changes in exposure along the flyway
Little is known about the behaviour of many migratory birds during their long-distance migrations. Critical resting sites are known for many species, but there is a lack of detailed studies of habitat use and behaviour at and around these sites, and hence a lack of knowledge of potential HPAI exposure, between individuals of the same species, individuals of different species, and between wild bird species and domestic ducks and poultry. The global nature of the disease and its extensive reach along migration flyways means that there is an urgent need to obtain more information on habitat use and behaviour across the world, so that more integrated international approaches to the surveillance and management of HPAI can be developed. Coupled with data from genomics studies, such studies could help differentiate further between these routes and their significance for HPAI transmission.
3.3.4 Changes in behaviour and susceptibility of birds resulting from wider environmental stresses.
Recent research has shown an association between the depletion of energy stores and reduced immuno-competence in a migratory passerine (Eikenaar and others 2020). There is a body of research that shows that petrochemical pollutant exposure in birds can result in prolonged immunosuppression (King and others 2021), and the prevalence of avian influenza in wild ducks has been shown to be associated with body mercury concentrations (Teitelbaum and others 2022). The potential impacts on sensitivity to HPAI through stresses caused by environmental changes such as warming seas and extreme weather, and anthropogenic changes such as offshore wind energy developments, are also not understood. These changes may have impacts on individuals and populations under certain circumstances, and further evidence on the interactions between these different factors, from both modelling and empirical approaches, would be valuable.
3.4 Management options to reduce risk to wild birds.
There are relatively few options for management directed at wild birds that can potentially reduce the impacts of HPAI on populations. The principal ones are vaccination of wild birds; supplementary or diversionary feeding; carcass removal; restrictions on release of birds for shooting, and reduction in wider anthropogenic and environmental stressors. These are described in the paragraphs below and summarised in the ‘Management options to reduce avian influenza risk to wild birds’ section. It should be noted that the evidence underpinning the specific management actions is sparse and there is uncertainty around their effectiveness, both in general terms and how success may vary according to population, disease, and local contexts.
3.4.1 Vaccination of wild birds
Vaccination of wild birds is a strategy that has been used previously to protect vulnerable populations from disease (for example, protection of albatross chicks from avian cholera (Bourret and others 2018). Evidence from captive wild birds indicates that vaccination can be effective in protecting individual birds against HPAI. Vaccination of Gyr-Saker falcons in captivity showed reduced mortality and viral shedding using inactivated H5N2 virus against H5N1 inoculation (Lierz and others 2007). Inactivated H5N2 has also been used in a zoo setting in France, to provide protection at an individual level for 25,000 birds across 126 species (Lécu and others 2009). That study showed that different avian orders reacted differently to the vaccine and suggested that a booster would be needed for vaccinated individuals every 6 to 12 months to maintain their protection, depending on the species. However the benefits of such vaccination are at the individual level, and not at the population level, since the vaccines are poorly matched and would require updating to deliver more widespread benefits.
Vaccination of wild birds in open environments would only be feasible if it could be done in young birds prior to fledging. Furthermore, the need for boosters with current vaccines to ensure continued protection means it would not generally be possible. There would also be considerable practical difficulties associated with the vaccination of wild birds, especially for colonies in remote locations. Nevertheless, even a relatively poor vaccine would provide vaccinated individuals with some protection from illness, even if it did not stop virus replication and shedding. While there are multiple challenges that would have to be addressed for deployment of vaccines to be practicable (see Section 4 below), vaccination of wild birds should remain under consideration as a last resort option, for example in a situation where a species was at risk of HPAI-related extinction.
3.4.2 Supplementary or diversionary feeding
Supplementary or diversionary feeding can reduce pressure from birds on certain areas or resources and has been used as a management strategy to reduce predation, for example from hen harriers on grouse (Ludwig and others 2018) and from kestrels on little terns (Smart and Amar 2018). Diversionary feeding has also been used to reduce grazing pressure and damage to agricultural crops by geese on Islay; Scotland (McKenzie and Shaw 2017).
In a similar fashion, supplementary or diversionary feeding could be used to manipulate the behaviour of predatory or scavenging birds to reduce their risk of exposure. However, there is a risk that diversionary feeding can encourage aggregation of birds in other areas, which may increase contact and risk of transmission among wild birds. As a result, this is not a management option that we would recommend.
3.4.3 Carcass removal
The Ramsar Wetland Disease Manual states in Section 3.4.2 that “Rapid and effectively planned carcase collection and disposal is essential to prevent spread of infectious disease” (Cromie and others 2012). During the 2022 outbreak in seabirds, carcass removal was employed at a range of seabird colonies in Great Britain and the Netherlands as a response to the emergency. In England, carcass removal was deployed daily at Coquet Island once extensive mortalities were occurring, and at Scolt Head Island. In the Netherlands, the extent of carcass removal was variable between Sandwich tern colonies and may have had some beneficial impact (Rijks and others 2021).
However, there is currently a lack of robust empirical evidence for the benefits of carcass removal for HPAI, and modelling suggests that it may only be beneficial in reducing infections within bird populations within a relatively small range of conditions (D. Haydon, unpublished). The process of carcass removal may also risk the spread of fomite and faecal contamination, as well as posing health and safety risks to operators. Nevertheless, carcass removal may be one of the only potential tools for effective mitigation of an active outbreak for residual and rare populations. As a result, HPAIG are supportive of further trials of carcass removals in circumstances with high mortality of wild birds to understand its efficacy and learn more about the practicalities of doing so.
3.4.4 Restrictions of the release of birds for shooting
There are existing regulations prohibiting the release of birds for game shooting within avian influenza restriction zones.
A recent Natural England (NE) evidence review concluded there was poor understanding of the potential role of released gamebirds in transmitting parasites and disease to native wildlife, as well as the wider ecological impacts of such releases (Madden and Sage 2020). Approximately 40% of gamebirds that are released in Great Britain are sourced in continental Europe as eggs or day-old chicks (Fujiwara and others 2022). The overall likelihood of HPAI virus introduction via hatching, egg movement and onward transmission into rearing sites is considered low, but there is considerable uncertainty around this, and this assessment assumes good compliance with biosecurity measures (Fujiwara and others 2022).
The high numbers of birds released (approximately 40 to 60 million captive-reared pheasants and red-legged partridges, and 2.5 million ducks annually) are a substantial minority compared with a total wild avifauna of 150 to 160 million. Of 2,714 H5N1 confirmed submissions of found dead birds from October 2021 to November 2022, 80 were from pheasants, the eighth highest category (Defra, unpublished data). Ducks are also at high risk of HPAI and can have the virus for several weeks before showing symptoms. Based on a qualitative risk assessment, a Defra report concluded that the risk of transmission of HPAI from gamebirds to certain wild bird groups, either from gamebirds infected prior to or after release, was high to very high (Anon. 2023). Gulls and birds of prey were at the highest risk of infection, but there was high to very high uncertainty around the high to very high risks predicted. The risk assessment suggested that the direct risks to seabirds from gamebirds infected with HPAI were negligible.
There is clearly considerable uncertainty around the risks of transmission to wild birds posed by gamebird and waterfowl releases. Nevertheless, the very high numbers of gamebirds that are released, almost doubling the biomass of the wild bird population over a short period of time, pose potential disease risks to other birds, not only as a direct source of transmission. They could also maintain HPAI in the other wild bird populations or increase infection pressure to resident wild birds over the late summer and early autumn, before migratory waterfowl species arrive in Great Britain in late autumn. Feeding stations provided for gamebirds could provide a high-risk environment for transmission if they artificially concentrate individuals beyond what would occur naturally, and there are also risks of onward transmission to predators, scavenging raptors and mammals, which may be attracted to these sites.
There is a need for further quantified work on the risk posed by gamebird releases. Modelling could be used to quantify the risks more precisely, and phylodynamic analysis would be useful in providing further insights into any role played by gamebirds in the current outbreak. Nevertheless, based on the range of evidence currently available, consideration should be given to minimise risks to wild birds by extending current bans on releases of gamebirds and waterfowl, which are currently limited to AI restriction zones, to include areas where HPAI infection risks are high, with potentially serious impacts on the conservation or welfare of wild birds.
3.4.5 Reduction in wider anthropogenic and environmental stressors
Since birds experiencing physiological and nutritional stress are more susceptible to infection and progression of disease, it is important that a strategy to combat avian influenza takes a holistic approach within a broader context of effective conservation and management of wild birds. This is especially important for seabirds, waterbirds, and raptors whose populations are vulnerable to HPAI. Although there is a large body of conservation legislation to protect and enhance bird species, many populations are in decline. It is therefore vital to enhance conservation initiatives that directly or indirectly benefit bird populations both onshore and offshore and take a precautionary approach regarding developments that may exacerbate the existing adverse impacts of stressors such as climate change, offshore wind farm development or overfishing.
Management options to reduce avian influenza risk to wild birds
Vaccination of wild birds
Benefits:
- may provide short to medium-term protection for a range of species
Comments:
- licensed vaccines with known characteristics in terms of efficacy and delivery for wild birds not currently available
- practically challenging at large scale and for remote colonies
Supplementary or diversionary feeding
Benefits:
- can reduce pressure from birds on certain areas or resources
- could reduce risk from wild birds to poultry premises
- can modify the behaviour of predatory or scavenging birds to reduce their risk of exposure
Comments:
- can encourage aggregation of birds in other areas, which could be likely to increase contact and risk of transmission among wild birds
- effective in reducing predation pressure and scavenging in certain situations, although its use and benefits in relation to reducing HPAI transmission have not been previously demonstrated
Carcass removal
Benefits:
- anecdotal accounts of some benefits when deployed at Coquet Island and Scolt Head Island
- variable success across different locations in the Netherlands
- some evidence that carcass removal at an early stage can reduce mortality of Sandwich terns due to HPAI
Comments:
- the process of carcass removal can cause disturbance, and potentially lead to abandonment and dispersal
- lack of consistent evidence regarding benefits
- modelling suggests that it may only be beneficial within a relatively small range of conditions
- local contexts including community perceptions also need to be considered
Extension of restrictions on release of birds for game shooting and wildfowling beyond disease restriction zones
Benefits:
- may reduce risk of released birds infecting wild birds or acting to maintain or amplify infection in birds, mammals, and the environment, especially in relation to gamebirds
Comments:
- high uncertainty around the level of risk, but likely to be higher for gamebirds than waterfowl due to very high numbers released
- concerns around how well existing biosecurity requirements are followed
Reduction in wider anthropogenic and environmental stressors
Benefits:
- reduction in physiological and nutritional stress of individual birds, making them less susceptible to infection
- reduction in population-level and site-specific threats, such as declines in food resources and pressures from offshore wind farm developments
Comments:
- lack of detailed understanding of how multiple stressors interact, and overall benefits of reducing individual stressors
3.5 Monitoring and surveillance
While the existing wild bird passive surveillance system for avian influenza provides useful information, it was not designed as a surveillance system to inform our understanding of risks and vulnerabilities of wild bird populations. It is primarily a sentinel system to inform our understanding of infection pressure and therefore the potential challenge to domestic poultry in particular parts of Great Britain and has shown that changes in wild bird infections precede poultry incursions. However, the existing surveillance system is insufficient to provide precise information on spatial and temporal patterns of HPAI infection, or to increase our understanding of why some species are vulnerable. Further, it is insufficient to provide early warnings of likely increases in virus prevalence or specific challenges to certain vulnerable populations.
Repeat monitoring is essential for providing information on patterns and trends in populations and their disease status, and to identify why some populations are vulnerable. Active surveillance for infection provides significant benefits over passive surveillance, including additional information on prevalence, but it requires sampling of live birds. In the Netherlands, over 180 bird ringers have been trained to take blood samples, swabs, ectoparasites and faeces, providing much improved sampling capacity. Surveillance in the Netherlands coordinated with these volunteers has been shown to be successful in detecting West Nile Virus in whitethroats (Sikkema and others 2020) and Usutu virus in wild blackbirds (Rijks and others 2016), although there is no specific evidence to date on the benefits of this system in detecting HPAI. Active surveillance that is sufficient to provide robust estimates of prevalence would require a substantial increase in sampling and testing capacity with likely high associated costs, and a cost-benefit analysis to inform decisions on investment as well as the most cost-effective design of such a system.
Active surveillance for infection normally requires blood samples, but current Home Office regulations mean that blood samples can only be taken by an individual holding a personal Home Office Licence linked to a project licence. A modification of the Home Office rules to allow blood sampling of birds by trained volunteers still adhering to strict ethical and welfare protocols, as has been adopted successfully in the Netherlands, would enable more comprehensive surveillance and enhance understanding of the disease. In the absence of this, BTO has the potential to license individuals for swabbing procedures with appropriate training, which could lead to a significant increase in sampling. However, increased blood sampling would be desirable since this enables testing for previous exposure to HPAI via antibodies, whereas testing of swabs is limited to identifying current infections. The increase in blood sampling that results could be substantial and may lead to current capacity in APHA laboratories being exceeded. Any modifications to blood sampling regulations would therefore also be likely to require licensing of new laboratories for testing, and, or the establishment of validated protocols for inactivation of potentially virus-containing samples in the field before analysis in Biosafety Level 2 (BSL2) laboratories. Appropriate standards would also be necessary to ensure equivalence of results with those from the National Research Laboratory at APHA.
4 Is vaccination a viable route forward?
4.1 Why vaccination should be considered
HPAI control in Great Britain currently relies on a combination of culling of flocks once avian influenza is identified, and enhanced biosecurity measures where there is a perceived elevated risk in Avian Influenza Protection, Monitoring and Surveillance Zones to augment routine biosecurity measures. Where high levels of biosecurity can be maintained, the risk of HPAI virus exposure is likely to be low. Conversely, low levels of biosecurity are often identifiable in premises where HPAI has been identified. However, pre-emptively identifying premises that are more likely to experience biosecurity lapses may be difficult. Effective incursion of HPAI may potentially arise from many different routes of exposure involving any of humans, kept birds and wildlife and potentially high levels of environmental contamination. The circumstances which result in effective transmission may also be highly transient and therefore difficult to both identify and prevent. Under these circumstances, biosecurity can become difficult to maintain, particularly at key points in production cycles. This includes, for example, thinning of broiler flocks (by the partial removal of flock populations to facilitate growth in the remainder). Even if the appropriate issues are identified, and physical risks reduced (for example, via improved housing, processes, and systems), this would be in the context of substantial uncertainties that accompany farming systems experiencing squeezed profit margins and substantial performance pressures. These conditions may also be exacerbated by issues generated by the HPAI epidemic itself, which make incursion more likely even in situations of relatively robust biosecurity. Research has highlighted how social, economic, and psychological factors in relation to farmers and farm staff affect levels of initial investment in and sustained adherence to biosecurity measures (Maye and Chan 2020). Biosecurity is also more difficult in parts of Great Britain’s highly differentiated poultry and poultry products markets which include free range produce as well as smaller, non-commercial holdings. If undertaken, increased biosecurity may entail changes to production systems, altering risk and cost profiles rather than removing risks entirely. Moreover, there may be greater environmental externalities associated with highly biosecure poultry production systems (Graham and others 2008, Hinchliffe and others 2013). Thus, while biosecurity is a key component in infection prevention and disease control, limitations in terms of coverage, compliance and environmental consequence make consideration of vaccination particularly relevant.
Such considerations must include the performance requirements for vaccination, which are at least partially determined by the strategic objectives of the disease control policy. For example, endemic circulation of HPAI in Great Britain wildlife, with a year-round risk of outbreaks in domestic poultry across a broad geographical range, presents a considerably different challenge to that experienced prior to 2021. In this case, disease eradication in poultry may be impossible, and so a vaccine that significantly reduces the impact of disease with even a low level of protection against infection may be acceptable if it reduces the overall cost and, or logistical burden of outbreaks. However, if a vaccine is expected to contribute to disease eradication with culling of infected poultry flocks, for example, when flock-to-flock spread is substantial, then a vaccine must provide a high level of protection against infection under field conditions, especially as low protection levels in this case may slow rates of spread without stopping it. This may increase the time to detection, potentially increasing the risk of onward spread (Nickbakhsh and others 2013).
Vaccination against viral diseases of poultry is well-established, as is the case for the economically important, notifiable, “Newcastle disease” where use of vaccines is routinely accepted as an important preventive control measure to be used in conjunction with biosecurity and culling (Dimitrov and others 2017). Thus, the logistical challenges of vaccination are not, in themselves, a barrier. However, there are additional challenges to the adoption of an HPAI vaccine that are not present for Newcastle disease. Strain variability of Newcastle disease is substantially less and there are no zoonotic risks, and so for example the use of any live virus vaccine carries a considerably lower regulatory burden. Nonetheless, a broad range of vaccines with proven benefit against AI under certain circumstances exist in Europe, though none have yet been marketed and approved that specifically protect against the current prevalent strains of H5N1. In principle, they could be developed and approved relatively quickly because they are based on either commonly used viral vector vaccines or based on inactivated AIV vaccines, so long as there is sufficient impetus and therefore commercial potential.
4.2 Characteristics of a useful vaccine
Effective vaccination in poultry must consider the relatively short lifespan of most kept birds, and the large populations with often relatively low per bird value. As such, useful vaccination is likely to be limited in scope. Following broadly accepted criteria (see Swayne and Simms 2020), a useful vaccine should ideally be:
- cost-effective and useable as part of ongoing vaccination program
- antigenically like the prevalent field viruses of interest
- effective in multiple avian species (while duck specific AIV vaccines have been developed in China, currently vaccines are mostly used in meat, layer, and breeder chickens, and in ducks, with relatively minor use in turkeys, geese, quail, and other species; (see Swayne and others 2011)
- administered via low-cost mass application methods
- a DIVA (“Differentiating Infected from Vaccinated Animal”) vaccine that is serologically distinguishable from infection (Capua and others 2004)
- applied at one day of age in hatchery or in ovo
- producing a protective humoral response in the presence of maternally derived antibodies (MDA)
It is extremely unlikely that vaccination against HPAI will eradicate disease risks. However, there is evidence that vaccination against HPAI has had some success when implemented as an additional control tool in many countries with endemic disease, including China, Vietnam, Indonesia, and Egypt (Swayne and others 2011, Swayne and Spackman 2013, Brown and others 2016). Additional vaccination programs for H5 or H7 AI vaccines have also been used as preventative measures against HPAI in 20 European and Asian countries in zoos, for hunting and companion birds, and for conservation of endangered captive birds.
4.3 Barriers to vaccination
Vaccination can provide a tool to reduce quantities of circulating virus and minimise the number of birds which need to be culled, thereby also addressing public concerns towards stamping out and protecting public health. However, barriers to vaccination must be overcome and some may take longer than others, including trade issues and availability of sufficient commercial vaccines. Non-acceptance by importing countries is largely based on concerns that HPAI can be masked due to vaccination. Concerns are also raised that available AIV vaccines are less effective because several antigenic divergent strains are prevalent in different geographic regions. Also, the performance of vaccines has typically not been optimal in birds with maternal derived antibodies, as is likely under field conditions (Kim and others 2010). The willingness of the industry, the extent of costs and other socio-economic parameters must be assessed, and vaccine producers need to be made aware that there is a viable market for their products if conditions are indeed favourable. Vaccination may also result in the emergence of immune-escape variants which will require updating to antigenically match the vaccines to the escape variants (Chang and others 2020). To be of practical benefit, the vaccine formulations should allow differentiation between the infected and the vaccinated birds (DIVA). This will facilitate monitoring of vaccinated flocks to prevent undisclosed infection that may allow the evolution of new virus strains that evade vaccine protection or pose a greater risk to human health. It will also provide assurance to trading partners that domestic poultry are free from infection.
However, it has been proven difficult to implement DIVA tests when inactivated whole virus vaccines are used. Subunit vaccines or viral vector-based vaccines could provide a DIVA solution. However, killed virus vaccines are also in use, for example for protection against other subtypes of AIV such as low pathogenicity H9, H7, and, or H6 should they become co-circulating in Great Britain, then the adoption of killed virus vaccines against these subtypes, would compromise DIVA tests, even when vectored or subunit vaccine formulations are used against HPAI H5 viruses. In this case, new improved DIVA assays would also need to be developed allowing differentiation between the different AIV subtype and their corresponding vaccine formulations.
4.4 The cost-benefit balance for vaccination
Globally, the costs associated with HPAI outbreaks are very high; HPAI threatens food security and sustainability and is a burden on the taxpayer and public. Thus even imperfect vaccines have the potential to play a role in disease control. For example, in China, vaccination against the H7N9 strain of HPAI was initiated in 2017 and, within two years, virus isolation from field samples was reduced by over 90%, with a substantial reduction in human cases as well (Shi and others 2023). While these impacts are to some extent lessened by the continuous challenge that virus evolution presents to vaccine efficacy, they are nonetheless dramatic. In British poultry, the increased infection pressures experienced in recent years due to H5N1, and the potential for sustained pressure on the poultry industry (either through endemic circulation in wild birds or continued high infection pressures from migratory birds with possibly continued exposure to an expanded range of infected wild bird species) provided added impetus to consider how these barriers might be overcome.
Whether or not HPAI vaccination is a viable strategy will also depend on a combination of the protection the vaccines provide, the risks that would be mitigated by vaccination and most importantly the long term expected trajectory of the epidemic. Vaccines could be deployed:
- in response to episodes of high incursion risks that may be limited geographically, and in time, if the risk to poultry is high, and the expected duration of that risk is low
- as an emergency response to outbreaks, to create buffer zones where farm-to-farm spread is expected to be a substantial risk either on its own, or in combination with localised high risks of incursions from wild birds
- as a routine measure with no set time limit for the cessation of vaccination, probably targeting industry sectors or geographical areas where incursion risks are highest and, or benefit is greatest. This is most likely if HPAI is endemic, and if infection pressure is continually high, by some combination of circulation in local wild bird populations, farm-to-farm spread and, or sufficiently regular incursions into the poultry population
In all cases, vaccination is more likely to be considered where the logistics of control are placing an undue strain on veterinary services, where the economic costs of outbreaks are unsustainable. A particular issue is increased risk of occupational zoonotic transmission, as was a likely impetus for an extensive vaccination campaign in 2017 against the H7N9 AIV strain in China (Zeng and others 2018). Determining appropriate targeting and “trigger conditions” for initiating vaccination is therefore a necessary step in planning for potential deployment.
Also important are the properties of the vaccine itself. Most importantly, a vaccine which is effective at preventing severe clinical signs may be less effective at reducing virus shedding and preventing secondary disease impacts such as reduced egg production and quality or reduced weight gain (Meng and others 2009). If so, such a vaccine would have at best limited utility, given the relatively low value of the birds, and the likelihood that substantial proportions of the poultry population, especially broilers, are likely to remain unvaccinated and therefore highly vulnerable to infection following exposure.
Experimental trials of HPAI vaccination are being undertaken in France, Italy and the Netherlands. These are likely to provide invaluable information for any implementation of vaccination in Great Britain and should form the basis for any further studies specific to Great Britain. Depending on the nature of the existing work, additional studies may be necessary to determine whether the birds carry maternally derived antibodies to the vaccine antigen and to establish the duration of immunity under field conditions, for example, to determine how many doses of vaccine would be required to maintain the protective immunity for commercial breeds. Only limited data are available to set a benchmark at what levels of immunity in vaccinated birds fully protects them from clinical disease and reduces the virus transmission chain within the vaccinated flocks. Therefore, experimental vaccine trials would be needed in target avian species having variable levels of immunity.
Field trials under Great Britain conditions will also be necessary, covering the main species of birds reared and considering the requirements of different management systems, including free range. Population models (see Section 5) of the impact of vaccination will provide valuable information, including to identify conditions for the deployment of vaccination as a supplement to current policies. These models will require additional information that will in part be obtained from field trials, including the time to develop immune protection in birds, and the estimated efficacy against susceptibility and transmissibility. Outputs should include measures of both requirements and impact, including the reduction in number of infected premises (IPs), impact on onward transmission risk and logistical requirements to support it.
5 What is the expected future trajectory of the outbreak (this year and beyond)?
5.1 Background - modelling in domesticated poultry and wild birds
Quantitative epidemiological models (mathematical, statistical and computational) are useful tools which can be used to both retrospectively analyse past infectious disease outbreaks and also explore possible future trajectories. They enable us to both explore hypotheses regarding the characteristics of disease outbreaks, and to formally organise data based on our understanding of the mechanisms of spread. When models are parameterised by fitting them to epidemiological and experimental or field trial data, they can be used to project the impact of different disease control approaches, and thus inform the design of effective approaches to intervention.
Models rely on a combination of an understanding of the processes governing transmission as well as data on the characteristics of the populations at risk, and individuals being affected. Such models must always make simplifying assumptions, while aiming to capture the critical processes governing spread. The development of these assumptions is a critical part of the scientific process and an exploration of them is often a source of scientific insight in themselves. While there are as yet no relevant published models of HPAI transmission in wild birds, models have already been developed that are suitable for analysing spread amongst poultry rearing premises and have either been developed for, or are easily adapted to, epidemics in Great Britain. These models use data identifying the locations of poultry premises and numbers of kept birds per premises, together with specified locations and timings of known outbreaks of H5N1, to statistically estimate epidemiological parameters and transmission rates (Jewell and others 2009, Hill and others 2017). Other models (Truscott and others 2007, Dent and others 2011) have been developed to explore the impact of different types of contact risks (for example, via company ownership, common use of slaughterhouses, or shared workers) on outbreak dynamics. All these models have been used to explore the impact of interventions.
Further work has been commissioned by Defra under its Animal Health Modelling Capacity and the flu-map project and this work is ongoing. Though these models are sensitive to biases in the data, in principle methods exist to try to correct for this, for example by identifying critical demographic features that must be recapitulated in models, such as the scale of spatial clustering as had been done with foot and mouth disease (FMD) models (Tildesley and others 2010) and by creating synthetic populations that fill the gaps, based on the characteristics identified from known farms (Nickbakhsh and others 2011).
All mechanisms of viral transmission between poultry premises (aerosol spread and fomite transmission, and to a lesser extent, the movement of infected poultry themselves) have a strong spatial dependence, with premises near to an IP broadly speaking having a greater risk compared to those that are farther away. Even explicit links via human activity are likely to have spatial dimensions (Dent and others 2008). Further, transmission from wild birds via deposition of infected faeces in the environment also requires spatial proximity and movement patterns. Thus the risk of initial infection of a poultry premises, and the potential for onward transmission between premises is highly heterogeneous and therefore requires a good understanding of the demographics of the poultry population. This includes both spatial variation and the network of potentially infectious contacts that underpins much of the risk of farm-to-farm spread.
5.2 Data for modelling
5.2.1 Population demographics
Data on poultry premises exist from the “Great Britain Poultry Register” (GBPR), which is the compulsory registration system for all keepers of 50 or more birds. Registration is voluntary for keepers of fewer than 50. This database records the location, species and numbers for all poultry and gamebirds and has been recently updated. The lack of data on small holdings (less than 50) means that a measure of outdoor exposure is missing. If we could estimate the proportion of small holders infected it would be possible to assess direct exposure risks. This could be estimated by a survey or by extrapolating from veterinary investigations around IPs for example, although it may still be subject to some biases (for example, if numbers of small holdings were correlated with risks to larger premises).
The initial risk to poultry from wild birds is informed by the distribution and local abundances of relevant wild bird species. Data on wild bird distributions has previously been compiled in the context of the 2006 H5N1 epidemic (Snow and others 2007) and relevant data are available to 2014, however there will have been considerable changes to habitat, local conditions, as well as to the underlying populations. Current work under the Flu-Map project aims to exploit the e-Birds project to identify wild bird flyways ((Link to e-Birds project). Other data sources such as radar imaging have also been used in other countries. Despite challenges in identifying species-specific signatures (Zaugg and others 2008) and difficulties in picking up signals from low-flying birds (such as coastal seabirds), with suitable long-term investment these data could provide valuable, low cost and high resolution data on dynamic bird movements (Nilsson and others 2018).
5.2.2 Network data
Data on companies, slaughterhouses and catching companies had previously been collected in 2007 in response to the last H5N1 epidemic. These characterised the contact properties of the poultry industry (Dent and others 2008) and identified the importance of patterns of activity in driving transmission (Dent and others 2011, Nickbakhsh and others 2013). However, these are now substantially out of date, in a sector characterised by increased levels of vertical integration, variable contracts between farms and retailers and processors, and management consolidation. Ongoing work in the FluMap project aims to generate a network dataset though it will not be comprehensive across Great Britain. Such data are crucial to establish the potential risk of transmission within the poultry industry and how the structure of the underlying network may influence that risk.
5.2.3 Biosecurity
Poor biosecurity is regularly highlighted as the key risk for incursion of avian influenza virus into poultry housing. Therefore, how biosecurity varies between premises is an important consideration in models that include premises-level risk of exposure (for example in a model where many farms have moderate infection pressure with equal biosecurity there would be a very different range of outcomes compared to one in which half the farms have excellent biosecurity and half maintain it very poorly, even though the average outcome may be identical). Thus, while variation in biosecurity can be explored via sensitivity analyses, a quantitative assessment of the variation in biosecurity is a valuable input into models. Obvious issues such as entry points in terms of built infrastructure, and poor processes (such as boot changes), are regularly noted in investigations following outbreaks. However, developing a systematic understanding of poor biosecurity would necessitate detailed and extended audits to identify critical lapses and pressure points. Some factors, such as the economically critical moment of thinning in broilers, are often identified as a key moment of enhanced risk (Allen and Lavau 2015). Other less routine issues, such as staff absence, time pressures if working multiple farms, and use of contract labour at production pinch points, are likely difficult to identify during one-off inspections, but may still be important (Hinchliffe and others 2013). Therefore, any statistically robust study would likely have to involve a very large number of premises, if it were to identify significant and important differences.
5.2.4 Epidemiological data
Measures of the incidence of new infections and the prevalence of infection are necessary to understand the patterns of HPAI spread. Current models are driven by disease notification data for poultry (which includes data on date of reporting of infection, premises location, number of birds on the premises, and number of infected birds). Information on past exposure is based on serological testing which identify the presence of antibodies against the virus (Li and others 2021). Diagnostic tests based on identifying the presence of virus itself (for example PCR tests (Spackman 2020)) provide data on the current levels of infection. These data allow us to estimate the number of new infections over time (disease incidence) and in combination with other data allow estimation as well of attack rates, force of infection and the reproduction number of the disease.
5.2.5 Viral whole genome sequencing and phylodynamics
Whole genome sequencing technology allows for the inexpensive generation of the entire genetic makeup of virus samples taken from infected individuals. This information allows us to reconstruct the phylogenetic or ‘family’ tree of the sampled viruses, showing how closely related they are. This relatedness, when cross-referenced to the time it takes for the virus to evolve and other information (for example, the time samples are taken, the locations and species), allows us to reconstruct important information about epidemiological processes (Kao and others 2014). The use of pathogen phylogenetic data to inform our understanding of transmission processes is now well established when considering both broad geographical scales (Lycett and others 2020) and also finer scale transmission (Campbell and others 2018). Examples from the veterinary sphere exist in evaluating FMD transmission dynamics (Morelli and others 2012, Lau and others 2015) and to characterise outbreaks of bovine tuberculosis (Rossi and others 2022). Such data would be invaluable for informing transmission models at both the national and local scales. It allows us, for example, to directly estimate incursion rates from outside geographical areas, or from intermediate species (see Figure 4) (Custom R code developed using ancestral character estimation in R package “ape”: Paradis E, Schliep K. ‘ape 5.0: an environment for modern phylogenetics and evolutionary analyses in R’ Bioinformatics, 2019, 35, 526-528; the Time scaled tree was made using R package “treedater” Volz E, Frost S, ‘Scalable relaxed clock phylogenetic dating’ Virus Evolution, 2017, Volume 3, Issue 2, July, vex025).
While H5N1 virus is isolated and sequenced for Great Britain outbreaks in poultry, currently only one isolate per IP is sequenced. If this could be increased, it would facilitate the exploitation of those methods to integrate sequence data with transmission, providing better estimates of incursion rates, and quantification of rates of within-premises and potentially farm-to-farm spread. Further, there is currently no systematic effort to gather such data in wild birds where they may reside in or utilise habitat near farms, and this would be invaluable for quantifying the interactions between wild and kept birds. It is acknowledged that, for some species such as gulls, interactions may be transient, (O’Hanlon and others 2022), but may yet play an important role, and so may require a considerable sampling effort.
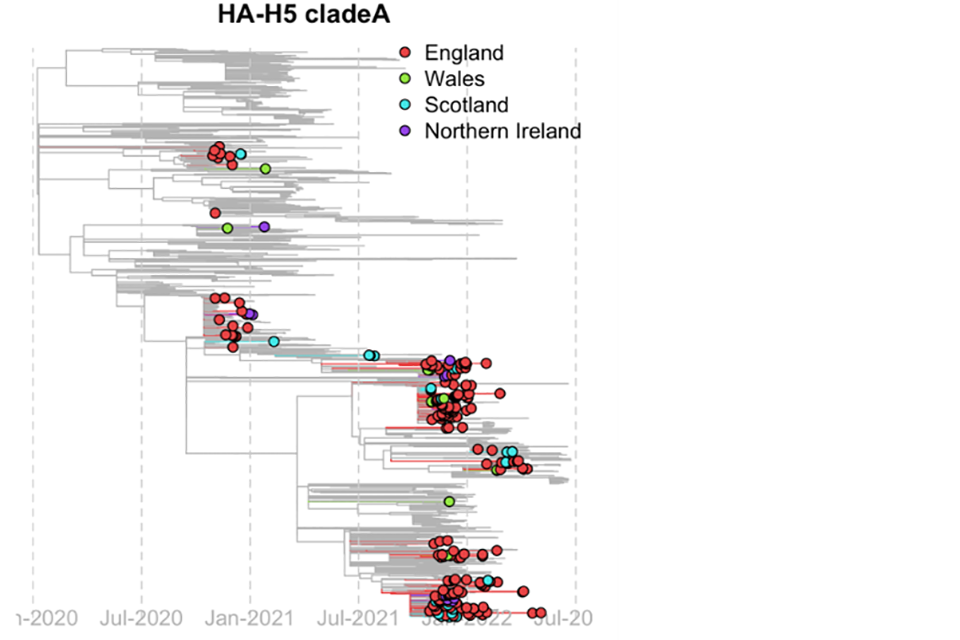
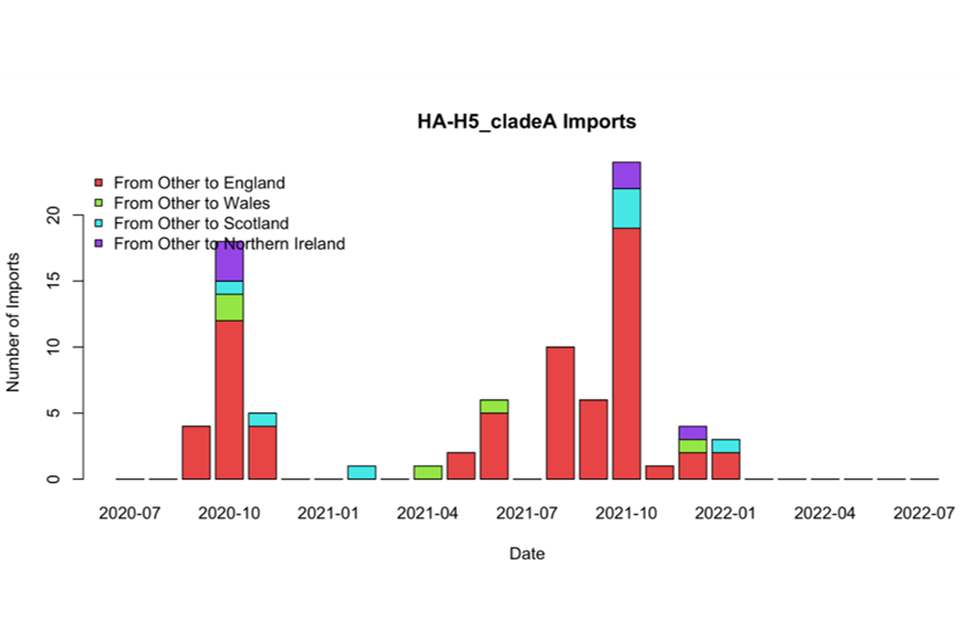
Figure 3: Reconstructed time-scaled phylogenetic tree based on sequences obtained from H5N1 virus obtained from wild bird samples taken to June 2022 (top) and estimated number of imports into each of England, Scotland, Wales and Northern Ireland, showing multiple imports across all seasons but with an estimated peak in October (bottom). Importation rates calculated using time scaled trees per Clade and discrete traits analyses. Estimates will be sensitive to sampling biases and model type (figure courtesy of Dr SJ Lycett, Roslin Institute, in work funded by the Flu-MAP project).
5.3 Models and uses
5.3.1 Models at national scales
Quantitative models at the national scale can be used to assess the overall impact of interventions and project future epidemic trajectories. Veterinary investigations currently suggest that the most substantial risk to premises is via the wild birds rather than from other farms. While this remains to be quantified, it is most likely that the proportion of IPs caused by wild birds is high and thus any national scale models must be informed by our knowledge of the risk from wild birds. Where risk of wild bird infection from farms is sufficiently small, the risk from wild birds to farms can be viewed as an external infection pressure and can be estimated using a combination of data analysis and statistical or computational models. Where circulation within poultry and, or between poultry and wildlife is substantial, models should consider both poultry and infection dynamics in wildlife simultaneously.
When combined with data, these models provide important information on which premises are most at risk of transmission. For example, Figure 4 compares the distribution of infection in premises to the recorded distribution of all premises, across four monthly periods from October 2021 to December 2022. All distributions show two peaks (are bimodal) however the peak is more pronounced in infected premises, compared to the entire population. The increase in the proportion of infections in large premises is especially marked in the October to December period, and this correlates in time, with the increase in incursions into Great Britain from elsewhere (Figure 4). However, there is also considerable local clustering of IPs (Figure 1). Models informed with sufficient data can be used to identify whether these are causally linked but would require a better understanding of local infection pressure from wild birds in areas surrounding the IPs (epidemiological data) and include both the potential impact of non-migratory wild species and the possibility of local transmission between premises (company and network data).
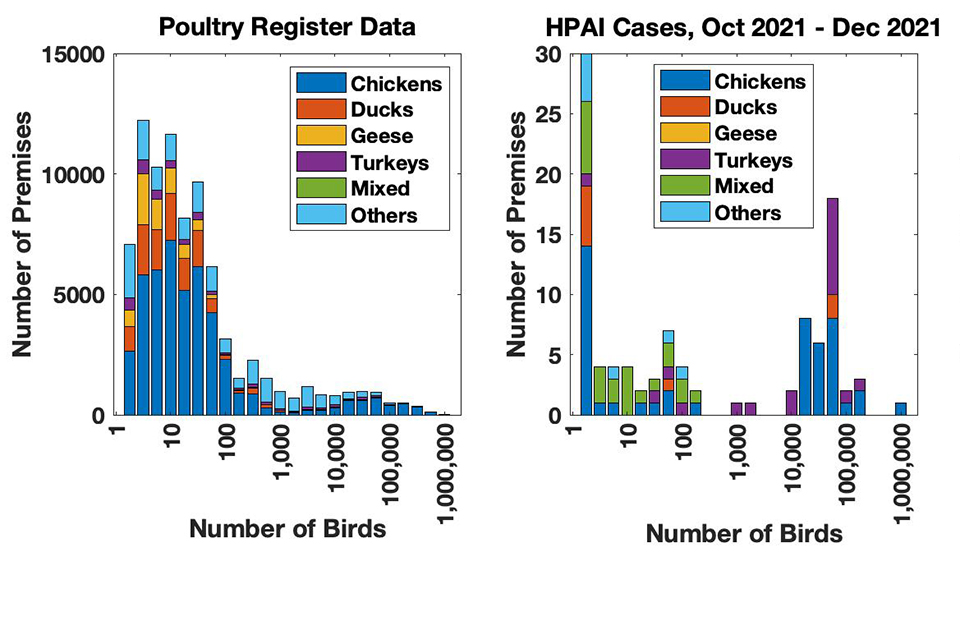
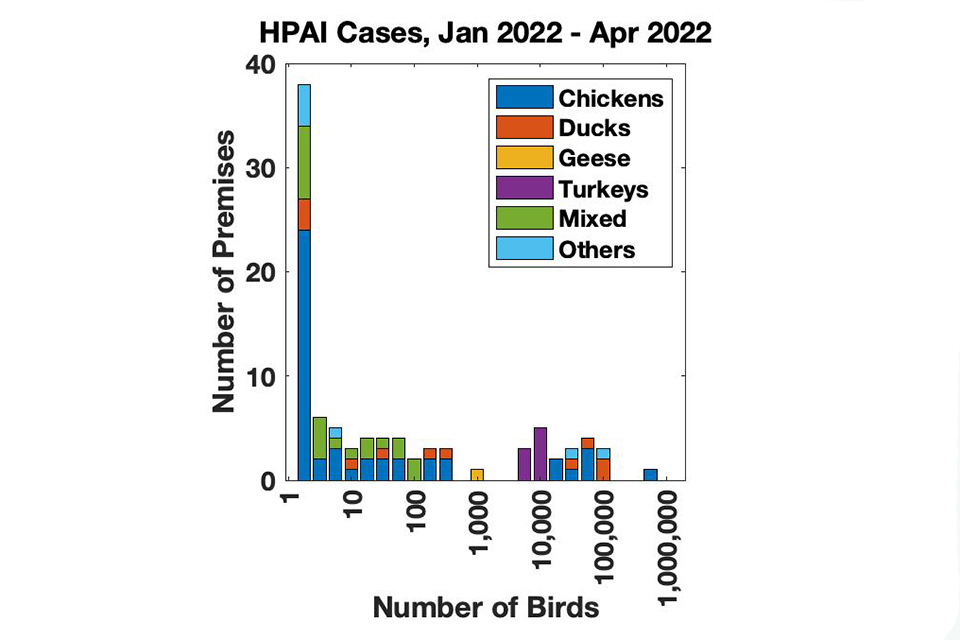
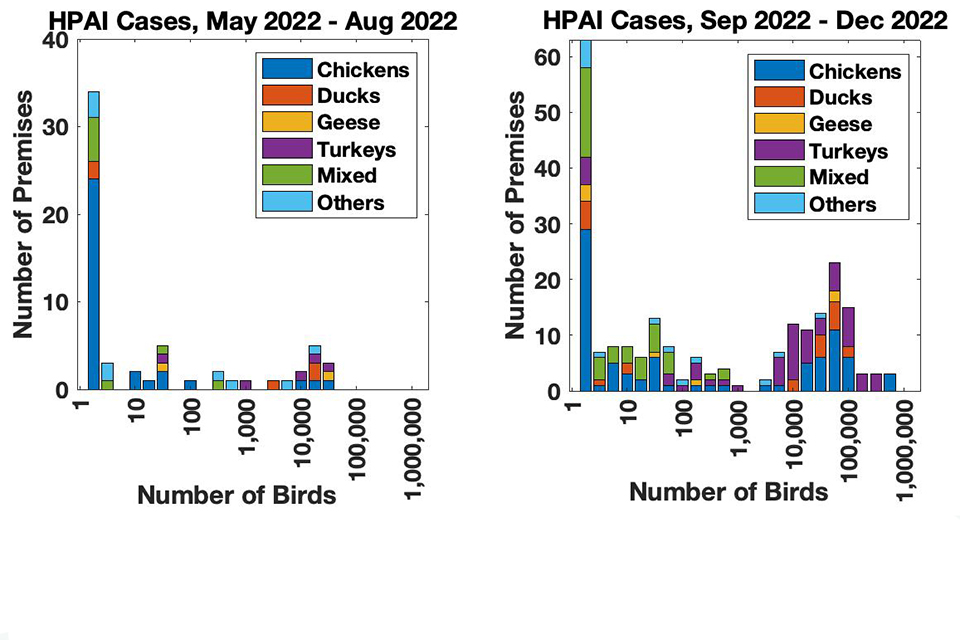
Figure 4: Distribution of premises in the GBPR and IPs by farm size and species (data extract as of 21 December 2022)
Figure 4 shows the distribution of all premises recorded in the Great Britain Poultry Register (GBPR), compared to the distribution of outbreaks of HPAI. These are differentiated by premises populations and recorded species. The comparison covers, across four separate panels, four time periods of October to December 2021, January to April 2022, May to August 2022, and September to December 2022. For the last period, data is truncated by the data extraction date of 21st of December 2022. The distribution of the number and type of infected premises appears to be markedly different in the autumn season, when there are many more infected premises with over 10,000 birds as the recorded population. The proportion of large premises where infected birds have been confirmed is also much higher than the proportion of all premises that are large, as recorded in the GBPR and as seen in the first panel. The effect is particularly marked in turkeys, though this may also reflect increases in turkey populations leading up to Christmas. In contrast, infected premises with recorded populations of less than 10,000, stays much more similar over other seasons in both years. The distribution of infected premises in the January to April and May to August periods, is also more similar to the distribution of all premises sizes recorded in the GBPR, though there still appears to be an elevated risk in larger premises in these time periods. As well, in the autumn period when the increase in the number of large infected premises is apparent, the rates of infection and incursions from outside GB as shown in Figure 3 are particularly high. GBPR premises are typically listed as possessing a single species, with the exception of those listed as “Mixed Backyard” premises. In this database, the typical stock size or overall number of birds is given, but this is not broken down by species. In the Infected Premises database, there are several instances where multiple infected species are reported on the same premises, suggesting that it may be necessary to investigate the species composition of premises that are reported in the GBPR.
5.3.2 Models at regional or local scales
Regional or local models can be used to forensically investigate single outbreaks or clusters of outbreaks, for example to assess whether the outbreak is consistent with farm-to-farm spread, or to quantify important disease parameters at the individual bird level (for example, the average transmission rate, or the time to mortality). When combined with wild bird data they can be used to better understand local circulation patterns and assess intervention strategies at the local scale. Analyses of more data-rich outbreaks can also be used to inform national scale analyses where not all farms have the same level of data.
5.3.3 Models for wild birds
Developing detailed models of the circulation of HPAI in wild birds offers many challenges above that for poultry - in particular the populations will be more varied and the available data will be significantly less dense and with greater underlying uncertainties and potential for bias. This combination of complicating factors of multiple, only partially observed species that may contribute to transmission to differing degrees, the relatively limited knowledge of past exposure to AIVs and nature of cross-immunity make future projections difficult. Nevertheless, approaches to consider how various combinations of these factors might play out in the future, such has been undertaken previously for COVID-19, for example (Kissler and others 2020), would provide valuable input for understanding the context of future actions.
Such models would ideally consider multiple species, adding to those uncertainties. Despite these issues, models of wild bird populations can be useful as a means of contributing to analyses of hypotheses regarding possible H5N1 circulation scenarios, identifying data requirements and as a strategic tool to explore scenarios of future epidemic trajectories. Such models must also be informed by information both on the wild bird populations (numbers, contact patterns and turnover) as well as by epidemiological and immunological data. Serological tests can theoretically identify past exposure to H5N1 and other AIVs (but interpretation is complex and a series of assays to dissect responses required), which are necessary to project future trajectories of HPAI in the wild bird populations. While it may be difficult to directly assess cross-protection, in previous studies immunoglobulin levels in blood have been tracked over time in mallards (Tolf and others 2013). Thus, in principle, neutralisation assays against a panel of H1-H16 could provide some indication of cross neutralisation, which is at least partially correlated to cross protection.
6 Recommendations
The HPAI situation in Great Britain is challenging in terms of its impact on both wild birds and domesticated poultry, gamebirds and zoological collections. Much of the challenge is underpinned by our still developing understanding of HPAI circulation in Great Britain wild birds. Most importantly we need to know whether it is already endemic or likely to become endemic in non-migrating birds, the long-term trajectory of disease incidence, and an estimate of the likelihood of farm-to-farm transmission in poultry. Many actions will only have impact in the longer term, however there are considerable steps that should be taken as soon as possible, especially where these have the potential to have an impact within the next few years. Any actions arising from these recommendations will have to be subject to an appropriate assessment of cost and benefit. In any such assessment, especially where these involve a better evidence base to understand baseline conditions, Defra is urged to consider all of these in the light of not just HPAI, and in terms of costs to poultry production, but the potential gains for addressing longer term challenges.
6.1 Understanding host range
The propensity for host species to become infected by HPAIV and infect others in natural settings is most comprehensively determined by epidemiological studies. However, these can be difficult and expensive, especially if the species of interest are difficult to sample from, or experience infection at a low prevalence. Experimental studies can be used to provide baseline data on potential for species that may play an important epidemiological role, and in combination with other information, such as likelihood of contributing to poultry infections, could be used to guide further field studies.
Recommendation 1
‘Defra to support data acquisition and research funding for new investigations to characterise host range’. This includes:
- Targeted surveillance of potential bridging species that may act as vectors of infection to poultry and wild birds species of concern.
- Functional (H5 HA-binding; Shelton 2011) and, or biochemical (lectin staining or mass spectrometry) studies of receptor distribution in histology sections from key wild bird species.
- Matching studies with previous H5 viruses, to identify signatures of possible host range changes in the Gs/Gd lineage.
- Sequencing and functional testing of ANP32 genes.
6.2 Priority work on wild birds
Much of the current system for HPAI surveillance is passive, and oriented around identifying risks to kept bird populations. While such approaches have proven their worth in the past, current circumstances present novel risks to wild bird populations and risks to kept birds that are enhanced in terms of intensity, and in geographical and seasonal range.
Recommendation 2
‘Defra to develop enhanced surveillance systems for HPAI where the aim is to better characterise circulation in wild birds, in order to monitor threats to the conservation of wild bird species and ongoing risks to kept birds.’ These enhancements should include:
- An improved system for carcass monitoring for key species and sites, alongside a system of live-bird sampling similar to the approach used in the Netherlands.
- Improved demographic data to track the impacts of HPAI on two other potentially vulnerable groups of species – wildfowl and raptors.
- Improved disease surveillance in wild birds, including increase in sampling in live birds and acquisition of more serological data.
Recommendation 3
‘Defra to enhance monitoring of wild bird populations where the aim is to better characterise the populations themselves’. This includes:
- Annual seabird surveys at affected sites to better quantify long impacts of HPAI and deliver more extensive annual data across a wider range of sites and species, and an increase in the number of populations with long-term ringing effort to monitor survival.
- Enhanced monitoring of wild bird populations to provide greater confidence in their population trajectories and their underlying demographic drivers, including a repeat of the winter gull survey from 2003 and 2004 to 2005 and 2006 (Burton and others 2013) to quantify changes in overall population abundance and distribution.
Recommendation 4
‘Defra to improve the evidence base around potential management interventions to reduce the risk of HPAI to wild birds.’ While there is limited scope for actions to prevent mortality in wild bird populations, possible options such as carcass removal should be trialled at the nearest opportunity. Consideration should be given to extending restrictions on release of birds for game shooting beyond disease restriction zones, and further work conducted to quantify the risk posed by gamebird releases to maintaining or amplifying infection in wild birds.
Recommendation 5
‘Defra to include, in relevant evaluations of environmental management developments, assessments of the potential impact of HPAI on vulnerable species, including robust monitoring protocols.’ HPAI threats to wild birds come in the context of a range of other challenges to these populations, including both natural threats and management-driven activities. HPAI is a global, cross-boundary disease, and migratory species act to transmit HPAI along migratory flyways. It is important to understand how the combination of HPAI and other stressors will act on bird populations. This will further require working with international partners to identify risks, anticipate future threats and work towards cross-boundary solutions.
6.3 Vaccination of poultry
Implementation of any new policies for control will carry with it a legislative and logistical burden. Evaluating the strategic value of doing so will be underpinned by any evidence on the sources of infection (migratory birds, domestic birds and kept birds presenting different risks and leading to different policies) the duration of that risk (both seasonally and year to year) and the expected number of outbreaks and their costs. Thus many of the critical recommendations relevant to the vaccination of poultry centre around gaining a better understanding of the drivers of circulation and the expected duration of risk. These recommendations are covered in other sections.
Further, any recommendations will depend on an understanding of the scope and relevance of current French, Italian and Dutch vaccination studies. These should be ascertained and, where appropriate, results consulted before undertaking any further trials in Great Britain. In the meantime however, it would be expected that any implementation in Great Britain will require country specific feasibility studies and field trials.
Recommendation 6
‘Defra to develop specifications for trials, identification of potential field trial sites, and commission modelling studies (including assessment of required trial size and duration) to support this, beginning as soon as possible’, including the considerations outlined in this section. Experimental studies that consider the impact of maternal antibodies and work done on commercial breeds should be considered if these are not covered by the existing work in the EU.
6.4 Modelling of future projections
Both because we are uncertain how long the current situation will continue to challenge British poultry and threaten wild bird species, there is an urgent requirement to maintain demographic data which are vital for developing robust projections of future HPAI risks.
National scale models based on outbreak data can provide estimates of national level risks of HPAI spread from premises-to-premises, including variation in regional risk. They would also inform decision-making regarding vaccination and vaccine characteristics (for example, they might identify substantial risks for premises-to-premises spread). They can also be used to estimate poultry losses and the pressure on veterinary services resulting from outbreaks given estimates of infection pressures from wild birds. However, to provide robust recommendations, better data are required on kept birds, including potentially infectious contacts between them.
Recommendation 7
‘Defra to enhance collection of kept bird demographic data recorded in the Great Britain Poultry Registry’, making it more representative of current population numbers and species mixes and adding in requirements to provide additional information on company and other associated links that are considered high risks for transmission of HPAI.
Local scale modelling of outbreak data can be used to interpret surveillance data around infected premises to provide estimates of rates of premises-to-premises transmission.
Recommendation 8
‘Defra to facilitate the acquisition of data to support the estimation of rates of between premises HPAI transmission.’ This includes wider application of Whole Genome Sequencing, surveys and high-resolution tracking of individual bird movements, particularly around poultry premises. Further analyses of existing tracking data (for example, of gulls) to better quantify fine-scale habitat use and movements would provide a quick-win to improve quantification of these risks. Targeted data acquisition and analysis of IPs and surrounding areas. Developing a quantitative assessment of transmission pathways around outbreaks will be crucial to targeting interventions including vaccination. This to include deep surveys of wild birds and potential host mammals (especially rodents) around IPs and in surrounding areas, including obtaining dense viral sequence data, sequencing of isolates from infected birds in areas around IPs, investigation of biosecurity, and where a potential risk pathway has been identified, experimental studies to assess susceptibility of species with potential roles.
While there are many challenges to modelling circulation in wild birds, wild bird modelling would provide a strategic overview of possible outcomes to support planning, but would need to be supported by enhanced data on the range of species that are most likely to be contributors to circulating and possibly maintaining it in Great Britain wild birds.
Recommendation 9
‘Defra to improve data acquisition to support modelling of future trajectories in wild bird species of interest.’ This will require a combination of improved passive surveillance across multiple wildlife species, and targeted active surveillance for the purposes of research. These data would support characterisation of the “immuno-epidemiological” profile of HPAI exposure or infection in Great Britain, and cross-neutralisation studies, to inform the modelling of potential trajectories of population immunity and mortality.
6.5 Supporting these recommendations with improved data acquisition capability
Recommendation 10
‘Defra to facilitate, where it is safe and feasible to do so, streamlined procedures for generation of HPAI data.’
Underpinning these recommendations would be the substantial adjustments to our underlying data generation capacity, which would have to be accomplished without compromising any statutory requirements of monitoring of HPAI. One stumbling block to enabling this rapid expansion of data generation is the current need for high biosecurity “SAPO4/ACDP3” (specified Animal Pathogens Orders (SAPO) 04 refers to disease-producing organisms which are either exotic or produce notifiable disease and have a high risk of spread from the laboratory. Advisory Committee on Dangerous Pathogens category 3 (ACDP3) refers to microorganism which may present a risk of spreading to the community, but effective prophylaxis or treatment may be available) containment for any laboratory work involving live virus due to the capacity for human infection. However, in principle it should be possible to inactivate virus in the field using established methods to allow nucleic acid-based surveillance outside of high containment facilities. Approval of protocols for field inactivation are under discussion and should be prioritised to allow for the relevant expansion of laboratory capacity for PCR testing and viral sequencing. Additional requirements would also include:
- the development or implementation of rapid serological tests, to assess past exposure of wildlife to H5N1 and other influenza viruses
- easing of access to investigation sites for researchers and streamlined requirements for training, to enable rapid upscale of capacity
- whole genome sequencing of as many virus isolates, as possible, particularly where these can be used to investigate localised clusters of infection and where particular bird species may play an important role in disease circulation
It is further recognised that emergent infection problems are likely to become less predictable and may become more frequent, as we face increased challenges from climate change, stresses in biodiversity and adopt new land management strategies to combat these. Understanding these emergent problems will require better baseline data for evaluating the impacts of these multiple changes, and to facilitate rapid decision-making when emergent problems arise.
Recommendation 11
‘As a guiding principle, Defra should consider that any solutions to the issues of laboratory and surveillance capacity should not only address the requirements for the current HPAI situation, but also be formulated with the expectation that exotic and emergent disease problems are likely to become more frequent and more intense in the future.’
Acknowledgements
Dr S.J. Lycett for figures on phylodynamic analysis. APHA, National Emergency Epidemiology Group (NEEG) for provision of and interpretation of data. Many thanks for the support from the Defra SAC secretariat.
Bibliography
Abdel-Moneim AS, Abdel-Ghany AE, Shany SAS. ‘Isolation and characterization of highly pathogenic avian influenza virus subtype H5N1 from donkeys’ Journal of Biomedical Science, 2010; 17(1): 25.
Agüero M, Monne I, Sánchez A, Zecchin B, and others. ‘Highly pathogenic avian influenza A(H5N1) virus infection in farmed minks, Spain, October 2022’ Eurosurveillance 2023 28(3): 2300001.
Allen J, Lavau S. ‘Just-in-Time’ Disease’ Journal of Cultural Economy, 2015, 8: 342-360.
Anonymous. ‘Highly pathogenic avian influenza H5N1 rapid risk assessment: Catching-up of wild gamebirds in winter 2022 to 2023’ London, Defra. 2023.
Belser JA, Barclay W, Barr I, Fouchier RAM, and others. ‘Ferrets as Models for Influenza Virus Transmission Studies and Pandemic Risk Assessments’ Emerging Infectious Diseases journal, 2018, 24(6): 965-971.
Bourret V, Gamble A, Tornos J, Jaeger A, and others. ‘Vaccination protects endangered albatross chicks against avian cholera’ Conservation Letters’ 2018, 11 (4): e12443.
Brown IH, Abolnik C, Garcia‐Garcia J, McCullough S, and others. ‘High pathogenicity avian influenza outbreaks since 2008 except multi‐continental panzootic of H5 goose/Guangdong Lineage viruses’ Animal Influenza. DE Swayne, Ames: Wiley, 2016, 248-270.
Brown JD, Stallknecht DE, Berghaus RD, Swayne DE. ‘Infectious and lethal doses of H5N1 highly pathogenic Avian influenza virus for house sparrows (Passer domesticus) and rock pigeons (Columbia livia)’ Journal of Veterinary Diagnostic Investigation, 2009, 21(4): 437-445.
Burton NHK, Banks AN, Calladine JR, Austin GE. ‘The importance of the United Kingdom for wintering gulls: population estimates and conservation requirements’ Bird Study, 2013, 60(1): 87-101.
Caliendo V, Lewis NS, Pohlmann A, Baillie SR, and others. ‘Transatlantic spread of highly pathogenic avian influenza H5N1 by wild birds from Europe to North America in 2021’ Scientific Reports, 2022, 12: 11729.
Campbell F, Strang C, Ferguson N, Cori A, Jombart T. ‘When are pathogen genome sequences informative of transmission events?’ PLoS Pathogens, 2018 14(2): e1006885.
Capua I, Cattoli G, Marangon S. ‘DIVA - A vaccination strategy enabling the detection of field exposure to avian influenza’ Control of Infectious Animal Diseases by Vaccination, 2004, 119: 229-233.
Chang PX, Sealy JE, Sadeyen JR, Bhat S, and others. ‘Immune Escape Adaptive Mutations in the H7N9 Avian Influenza Hemagglutinin Protein Increase Virus Replication Fitness and Decrease Pandemic Potential’ Journal of Virology, 2020, 94(19): e00216-20.
Chauhan RP, Gordon ML. ‘A systematic review of influenza A virus prevalence and transmission dynamics in backyard swine populations globally’ Porcine Health Management, 2022, 8: 10.
Church GE, Furness RW, Tyler G, Gilbert L, Votier SC. ‘Change in the North Sea ecosystem from the 1970s to the 2010s: great skua diets reflect changing forage fish, seabirds, and fisheries’ ICES Journal of Marine Science, 2018, 76(4): 925-937.
Cromie RL, Lee R, Reeves R, Stroud DA. ‘Ramsar Wetland Disease Manual: Guidelines for Assessment, Monitoring and Management of Animal Disease in Wetlands’ Wildfowl & Wetlands Trust (WWT), 2012, Ramsar Technical Report No. 7.
Costa T, Chaves AJ, Valle R, Darji A, and others. ’Distribution patterns of influenza virus receptors and viral attachment patterns in the respiratory and intestinal tracts of seven avian species’ Veterinary Research, 2012, 43(1): 28.
Cummings CO, Hill NJ, Puryear WB, Rogers B, and others. ‘Evidence of Influenza A in Wild Norway Rats (Rattus norvegicus) in Boston, Massachusetts’ Frontiers in Ecology and Evolution, 2019, 7: 36.
Dent JE, Kao RR, Kiss IZ, Hyder K, Arnold M. ‘Contact structures in the poultry industry in Great Britain: exploring transmission routes for a potential avian influenza virus epidemic’ BMC Veterinary Research, 2008, 4: 27.
Dent JE, Kiss IZ, Kao RR, Arnold M. ‘The potential spread of highly pathogenic avian influenza virus via dynamic contacts between poultry premises in Great Britain’ BMC Veterinary Research, 2011, 7: 59.
Dhingra MS, Artois J, Dellicour S, Lemey P, and others. ‘Geographical and Historical Patterns in the Emergences of Novel Highly Pathogenic Avian Influenza (HPAI) H5 and H7 Viruses in Poultry’ Frontiers in Veterinary Science, 2018, 5: 84.
Dierschke V, Furness RW, Garthe S. ‘Seabirds and offshore wind farms in European waters: Avoidance and attraction’ Biological Conservation, 2016, 202: 59-68.
Dimitrov KM, Afonso CL, Yu QZ, Miller PJ. ‘Newcastle disease vaccines-A solved problem or a continuous challenge?’ Veterinary Microbiology 2017, 206: 126-136.
EFSA. ‘Avian influenza overview June – September 2022’ EFSA Journal, 2022, 20(10): 7597.
EFSA. ‘Avian influenza overview September – December 2022’ EFSA Journal, 2023, 21(1): 7786.
Eikenaar C, Hegemann A, Packmor F, Kleudgen I, Isaksson C. ‘Not just fuel: energy stores are correlated with immune function and oxidative damage in a long-distance migrant’ Current Zoology, 2020, 66(1): 21-28.
Floyd T, Banyard AC, Lean FZX, Byrne AMP, and others. ‘Encephalitis and Death in Wild Mammals at a Rehabilitation Center after Infection with Highly Pathogenic Avian Influenza A(H5N8) Virus, United Kingdom’ Emerging Infectious Diseases, 2021, 27(11): 2856-2863.
Fourment M, Darling AE, Holmes EC. ‘The impact of migratory flyways on the spread of avian influenza virus in North America’ BMC Evolutionary, 2017, Biology, 17(1): 118.
Franca M, Stallknecht DE, Howerth EW. ‘Expression and distribution of sialic acid influenza virus receptors in wild birds’ Avian Pathology, 2013, 42(1): 60-71.
Fujiwara M, Auty H, Brown I, Boden L. ‘Assessing the Likelihood of High Pathogenicity Avian Influenza Incursion Into the Gamebird Sector in Great Britain via Designated Hatcheries’ Frontiers in Veterinary Science, 2022, 9: 877197.
Furness RW, Wade HM, Masden EA. ‘Assessing vulnerability of marine bird populations to offshore wind farms’ Journal of Environmental Management, 2013, 119: 56-66.
Galbraith CA, Jones T, Kirby J, Mundkur T. ‘A review of migratory bird flyways and priorities for management’ CMS Technical Series, 2014, No. 27. Bonn, Germany. UNEP / CMS Secretariat.
Gamarra-Toledo V, Plaza PI, Inga G, Gutiérrez R, and others. ‘First Mass Mortality of Marine Mammals Caused by Highly Pathogenic Influenza Virus (H5N1) in South America’ BioRxiv, 2023, (preprint).
Graham JP, Leibler JH, Price LB, Otte JM, and others. ‘The animal-human interface and infectious disease in industrial food animal production: Rethinking biosecurity and biocontainment’ Public Health Reports, 2008, 123(3): 282-299.
Hamed MI, Amen OAK, Rateb HZ. ‘Detection of Avian Influenza Virus H5N1 in Horses at Assiut Governorate Egypt’ Journal of Advanced Veterinary Research, 2014, 4: 161-165.
Hill EM, House T, Dhingra MS, Kalpravidh W, and others. ‘Modelling H5N1 in Bangladesh across spatial scales: Model complexity and zoonotic transmission risk’ Epidemics, 2017, 20: 37-55.
Hinchliffe S, Allen J, Lavau S, Bingham N, Carter S. ‘Biosecurity and the topologies of infected life: from borderlines to borderlands’ Transactions of the Institute of British Geographers, 2013, 38: 531-543.
Jewell CP, Kypraios T, Christley RM, Roberts GO. ‘A novel approach to real-time risk prediction for emerging infectious diseases: a case study in Avian Influenza H5N1’Preventive Veterinary Medicine Journal, 2009, 91(1): 19-28.
JNCC. ‘Seabird population trends and causes of change: 1986–2019’ Peterborough, UK, Joint Nature Conservation Committee, 2021.
Kao RR, Haydon DT, Lycett SJ, Murcia PR. ‘Supersize me: how whole-genome sequencing and big data are transforming epidemiology’ Trends in Microbiology, 2014, 22(5): 282-291.
Kaplan BS, Torchetti MK, Lager KM, Webby RJ, Vincent AL. ‘Absence of clinical disease and contact transmission of HPAI H5NX clade 2.3.4.4 from North America in experimentally infected pigs’ Influenza and Other Respiratory Viruses, 2017, 11(5): 464-470.
Kim JK, Kayali G, Walker D, Forrest HL, and others. ‘Puzzling inefficiency of H5N1 influenza vaccines in Egyptian poultry’ Proceedings of the National Academy of Sciences of the United States of America, 2010, 107(24): 11044-11049.
King MD, Elliott JE, Williams TD. ‘Effects of petroleum exposure on birds: A review’ Science of the Total Environment, 2021, 755.
Kissler SM, Tedijanto C, Goldstein E, Grad YH, Lipsitch M. ‘Projecting the transmission dynamics of SARS-CoV-2 through the postpandemic period’ Science, 2020, 368(6493): 860-868.
Kuiken T, Rimmelzwaan G, Riel D, Amerongen G, and others. ‘Avian H5N1 influenza in cats’ Science, 2004, 306(5694): 241-241.
Langley LP, Bearhop S, Burton NHK, Banks AN, and others. ‘GPS tracking reveals landfill closures induce higher foraging effort and habitat switching in gulls’ Movement Ecology, 2021, 9: 56.
Lau MS, Marion G, Streftaris G, Gibson G. ‘A Systematic Bayesian Integration of Epidemiological and Genetic Data’ PLOS Computational Biology, 2015, 11(11): e1004633.
Le Gall-Ladeveze C, Guinat C, Fievet P, Vollot B, and others. ‘Quantification and characterisation of commensal wild birds and their interactions with domestic ducks on a free-range farm in southwest France’ Scientific Reports, 2022, 12: 9764.
Lécu A, De Langhe C, Petit T, Bernard F, Swam H. ‘Serologic Response and Safety to Vaccination against Avian Influenza Using Inactivated H5n2 Vaccine in Zoo Birds’ Journal of Zoo and Wildlife Medicine, 2009, 40(4): 731-743.
Lee DH, Bertran K, Kwon JH, Swayne DE. ‘Evolution, global spread, and pathogenicity of highly pathogenic avian influenza H5Nx clade 2.3.4.4’ Journal of Veterinary Science, 2017, 18(S1): 269-280.
Lee K, Lee EK, Lee H, Heo GB, and others. ‘Highly Pathogenic Avian Influenza A(H5N6) in Domestic Cats, South Korea’ Emerging Infectious Diseases, 2018, 24(12): 2343-2347.
Li Y, Ye H, Liu M, Song S, and others. ‘Development and evaluation of a monoclonal antibody-based competitive ELISA for the detection of antibodies against H7 avian influenza virus’ BMC Veterinary Research, 2021, 17(1): 64.
Lierz M, Hafez HM, Klopfleisch R, Luschow D, and others. ‘Protection and virus shedding of falcons vaccinated against highly pathogenic avian influenza a virus (H5N1)’ Emerging Infectious Diseases, 2007, 13(11): 1667-1674.
Long JS, Idoko-Akoh A, Mistry B, Goldhill D, and others. ‘Species specific differences in use of ANP32 proteins by influenza A virus’ Elife, 2019, 8: e45066.
Long JS, Mistry B, Haslam SM, Barclay WS. ‘Host and viral determinants of influenza A virus species specificity’ Nature Reviews Microbiology, 2019, 17(2): 67-81.
Ludwig SC, McCluskie A, Keane P, Barlow C, and others. ‘Diversionary feeding and nestling diet of Hen Harriers Circus cyaneus’ Bird Study, 2018, 65(4): 431-443.
Lycett SJ, Duchatel F, Digard P. ‘A brief history of bird flu’ Philosophical Transactions of the Royal Society B, 2019, 374(1775): 20180257.
Lycett SJ, Pohlmann A, Staubach C, Caliendo V, and others. ‘Genesis and spread of multiple reassortants during the 2016/2017 H5 avian influenza epidemic in Eurasia’Proceedings of the National Academy of Sciences of the United States of America, 2020, 117(34): 20814-20825.
Ma W, Kahn RE, Richt JA. ‘The pig as a mixing vessel for influenza viruses: Human and veterinary implications’ Journal of Molecular and Genetic Medicine, 2008, 27(3): 158-166.
Maas R, Tacken M, Ruuls L, Koch G, and others. ‘Avian influenza (H5N1) susceptibility and receptors in dogs’ Emerging Infectious Diseases, 2007, 13(8): 1219-1221.
Madden JR, Sage RB. ‘Ecological consequences of gamebird releasing and management on lowland shoots in England: A review by rapid evidence assessment for Natural England and the British Association of Shooting and Conservation’ North England. Peterborough. 2020, NEER016.
Martin G, Becker DJ, Plowright RK. ‘Environmental Persistence of Influenza H5N1 Is Driven by Temperature and Salinity: Insights From a Bayesian Meta-Analysis’ Frontiers in Ecology and Evolution, 2018, 6: 131.
Mastrandrea MD, Field CB, Stocker TF, Edenhofer O, and others. ‘Guidance note for lead authors of the IPCC fifth assessment report on consistent treatment of uncertainties’Intergovernmental Panel on Climate Change (IPCC), 2010.
Matilla A, Radrizzani M. ‘The Anp32 family of proteins containing leucine-rich repeats’ Cerebellum, 2005, 4(1): 7-18.
Maye D, Chan KW. ‘On-farm biosecurity in livestock production: farmer behaviour, cultural identities and practices of care’ Emerging Topics in Life Sciences, 2020, 4(5): 521-530.
McKenzie R, Shaw JM. ‘Reconciling competing values placed upon goose populations: The evolution of and experiences from the Islay Sustainable Goose Management Strategy’ Ambio, 2017, 46: 198-209.
Mehle A , Doudna JA. ‘Adaptive strategies of the influenza virus polymerase for replication in humans’ Proceedings of the National Academy of Sciences of the United States of America, 2009, 106(50): 21312-21316.
Meng D, Hui Z, Yang JM, Yuan JL, and others. ‘Reduced Egg Production in Hens Associated with Avian Influenza Vaccines and Formalin Levels’ Avian Diseases, 2009, 53(1): 16-20.
Mitchell I, Daunt F, Frederiksen M, Wade K. ‘Impacts of climate change on seabirds, relevant to the coastal and marine environment around the UK’ MCCIP Science Review 2020, MCCIP, 2020, 382-399.
Morelli MJ, Thebaud G, Chadoeuf J, King DP, and others. ‘A Bayesian inference framework to reconstruct transmission trees using epidemiological and genetic data’ PLoS Comput Biol PLOS Computational Biology, 2012, 8(11): e1002768.
Nickbakhsh S, Matthews L, Bessell PR, Reid SWJ, Kao RR (2011). ‘Generating social network data using partially described networks: an example informing avian influenza control in the British poultry industry’ BMC Veterinary Research, 2011, 7: 66.
Nickbakhsh S, Matthews L, Dent JE, Innocent GT, and others. ‘Implications of within-farm transmission for network dynamics: consequences for the spread of avian influenza’ Epidemics, 2013, 5(2): 67-76.
Nilsson C, Dokter AM, Schmid B, Scacco M, and others. ‘Field validation of radar systems for monitoring bird migration’ Journal of Applied Ecology, 2018, 55(6): 2552-2564.
O’Hanlon NJ, Thaxter CB, Burton NHK, Grant D, and others. ‘Habitat Selection and Specialisation of Herring Gulls During the Non-breeding Season’ Frontiers in Marine Science, 2022, 09: 816881.
Oswald SA, Bearhop S, Furness RW, Huntley B, Hamer KC. ‘Heat stress in a high-latitude seabird: effects of temperature and food supply on bathing and nest attendance of great skuas Catharacta skua’ Journal of Avian Biology, 2008, 39(2): 163-169.
Pavon-Jordan D, Clausen P, Dagys M, Devos K, and others. ‘Habitat- and species-mediated short- and long-term distributional changes in waterbird abundance linked to variation in European winter weather’ Diversity and Distributions, 2019, 25(2): 225-239.
Pearce-Higgins JW. ‘Climate Change and the UK’s Birds’ British Trust for Ornithology, 2021.
Perkins A, Ratcliffe N, Suddaby D, Ribbands B, and others. ‘Combined bottom-up and top-down pressures drive catastrophic population declines of Arctic skuas in Scotland’ Journal of Animal Ecology, 2018, 87(6): 1573-1586.
Puryear WB, Keogh M, Hill N, Moxley J, and others. ‘Prevalence of influenza A virus in live-captured North Atlantic gray seals: a possible wild reservoir’ Emerging Microbes and Infections, 2016, 5(8): e81.
Ramey AM, Hill NJ, DeLiberto TJ, Gibbs SEJ, and others. ‘Highly pathogenic avian influenza is an emerging disease threat to wild birds in North America’ Journal of Wildlife Management, 2022, 86: e22171.
Rijks J, Kik M, Slaterus R, Foppen R, and others. ‘Widespread Usutu virus outbreak in birds in the Netherlands, 2016’ Eurosurveillance, 2016, 21(45): 2-7.
Rijks JM, Hesselink H, Lollinga P, Wesselman R, and others. ‘Highly Pathogenic Avian Influenza A(H5N1) Virus in Wild Red Foxes, the Netherlands, 2021’ Emerging Infectious Diseases, 2021, 27(11): 2960-2962.
Root JJ, Bosco-Lauth AM, Marlenee NL, Bowen RA. ‘Cottontail rabbits shed Glade 2.3.4.4 H5 highly pathogenic avian influenza A viruses’ Archives of Virology, 2018, 163(10): 2823-2827.
Rossi G, Crispell J, Brough T, Lycett SJ, and others. ‘Phylodynamic analysis of an emergent Mycobacterium bovis outbreak in an area with no previously known wildlife infections’ Journal of Applied Ecology, 2022, 59: 210-222.
Shi J, Zeng X, Cui P, Yan C, Chen H. ‘Alarming situation of emerging H5 and H7 avian influenza and effective control strategies’ Emerg Microbes Infect Emerging Microbes and Infections, 2023, 12(1): 2155072.
Shriner SA, Root JJ. ‘A Review of Avian Influenza A Virus Associations in Synanthropic Birds’ Viruses-Basel, 2020, 12(11): 1209.
Sikkema RS, Schrama M, van den Berg T, Morren J, and others. ‘Detection of West Nile virus in a common whitethroat (Curruca communis) and Culex mosquitoes in the Netherlands, 2020’ Eurosurveillance, 2020, 25(40): 2-7.
Smart J, Amar A. ‘Diversionary feeding as a means of reducing raptor predation at seabird breeding colonies’ Journal for Nature Conservation, 2018, 46: 48-55.
Snow LC, Newson SE, Musgrove AJ, Cranswick PA, and others. ‘Risk-based surveillance for H5N1 avian influenza virus in wild birds in Great Britain’Veterinary Record, 2007, 161(23): 775-781.
Songserm T, Amonsin A, Jam-On R, Sae-Heng N, and others. ‘Fatal avian influenza A H5N1 in a dog’ Emerging Infectious Diseases, 2006, 12(11): 1744-1747.
Spackman E. ‘Avian Influenza Virus Detection and Quantitation by Real-Time RT-PCR’ Methods Mol Bio Methods in Molecular Biology, 2020, 2123: 137-148.
Sreenivasan CC, Thomas M, Kaushik RS, Wang D, Li F. ‘Influenza A in Bovine Species: A Narrative Literature Review’ Viruses-Basel, 2019, 11(6): 561.
Swayne DE, Pavade G, Hamilton K, Vallat B, Miyagishima K. ‘Assessment of national strategies for control of high-pathogenicity avian influenza and low-pathogenicity notifiable avian influenza in poultry, with emphasis on vaccines and vaccination’ Revue Scientifique Et Technique-Office International Des Epizooties, 2011, 30(3): 839-870.
Swayne DE, Simms L, Metwally S, Viljoen G, Idrissi AE. ‘Veterinary Vaccines: Principles and Applications’Food and Agriculture Organisation (FAO), Wiley Blackwell, 2020.
Swayne DE, Spackman E. ‘Current Status and Future Needs in Diagnostics and Vaccines for High Pathogenicity Avian Influenza’ Vaccines and Diagnostics for Transboundary Animal Diseases, 2013, 135: 79-94.
Teitelbaum CS, Ackerman JT, Hill MA, Satter JM, and others. ‘Avian influenza antibody prevalence increases with mercury contamination in wild waterfowl’ Proceedings of the Royal Society B-Biological Sciences, 2022, 289(1982).
Tildesley MJ, House TA, Bruhn MC, Curry RJ, and others. ‘Impact of spatial clustering on disease transmission and optimal control’Proceedings of the National Academy of Sciences of the United States of America, 2010, 107(3): 1041-1046.
Tolf C, Latorre-Margalef N, Wille M, Bengtsson D, and others. ‘Individual variation in influenza A virus infection histories and long-term immune responses in Mallards’ PLoS One, 2013, 8(4): e61201.
Truscott J, Garske T, Chis-Ster I, Guitian J, and others. ‘Control of a highly pathogenic H5N1 avian influenza outbreak in the GB poultry flock’ Proceedings of the Royal Society B: Biological Sciences, 2007, 274(1623): 2287-2295.
Velkers FC, Blokhuis SJ, Kroeze EJBV, Burt SA. ‘The role of rodents in avian influenza outbreaks in poultry farms: a review’ Veterinary Quarterly, 2017, 37(1): 182-194.
Verhagen JH, Eriksson P, Leijten L, Blixt O, and others. ‘Host Range of Influenza A Virus H1 to H16 in Eurasian Ducks Based on Tissue and Receptor Binding Studies’ Journal of Virology, 2021, 95(6): e01873-20.
Worobey M, Han GZ, Rambaut A. ‘A synchronized global sweep of the internal genes of modern avian influenza virus’ Nature, 2014, 508(7495): 254-257.
Yamamoto Y, Nakamura K, Yamada M, Mase M. ‘Pathogenesis in Eurasian Tree Sparrows Inoculated with H5N1 Highly Pathogenic Avian Influenza Virus and Experimental Virus Transmission from Tree Sparrows to Chickens’ Avian Diseases, 2013, 57(2): 205-213.
Yoon SW, Webby RJ, Webster RG. ‘Evolution and Ecology of Influenza A Viruses’ Influenza Pathogenesis and Control - Vol I, 2014, 385: 359-375.
Zaugg S, Saporta G, Van Loon E, Schmaljohann H, Liechti F. ‘Automatic identification of bird targets with radar via patterns produced by wing flapping’ Journal of the Royal Society Interface, 2008, 5(26): 1041-1053.
Zeng XY, Tian GB, Shi JZ, Deng GH, and others. ‘Vaccination of poultry successfully eliminated human infection with H7N9 virus in China’ Science China-Life Sciences, 2018, 61(12): 1465-1473.
Zhao CK, Pu J. ‘Influence of Host Sialic Acid Receptors Structure on the Host Specificity of Influenza Viruses’ Viruses-Basel, 2022, 14(10): 2141.
Annex A – Conflict of interest
The following conflicts of interest were declared by HPAIG members.
Rowland Kao
Recipient of UKRI and Defra funding under the “FluMap” project led by Dr. Ian Brown at APHA.
A member of the MoDIL (Modelling of Diseases Infecting Livestock) Consortium, which forms part of Defra’s Animal Health Modelling Capacity and has been the recipient of Defra funding for HPAI responsive analysis.
Neil Ferguson
Receives research funding from MRC for the Centre for Global Infectious Disease Analysis, from NIHR for the Health Protection Research Unit for Modelling and Health Economics, and from Community Jameel for the Abdul Latif Jameel Institute for Disease and Emergency Analytics.
Wendy Barclay
Recipient of UKRI and Defra funding under the “FluMap” project led by Dr. Ian Brown at APHA.
Paul Digard
Recipient of UKRI and Defra funding under the “FluMap” project led by Dr. Ian Brown at APHA.
Consultancy agreement with Freshfields LLC in the area of human influenza vaccines.
Piran White
Works on the York and North Yorkshire Natural Catchment Flood Risk Solutions project. I am the Principal Investigator on the University of York’s contribution to this project, which is led overall by City of York Council. The project is one of a number of projects funded within the UK government’s Flood and Coastal Resilience Innovation Programme (FCRIP), which is part of the government’s National Flood and Coastal Erosion Risk Management Strategy for England. FCRIP is funded by Defra and managed by the Environment Agency.” The value of the project to the University of York is £422,307.
I am also a member of Natural England’s Science Advisory Committee (NESAC).
Mike Tildesley
Member of the MoDIL Consortium, which forms part of Defra’s Animal Health Modelling Capacity and has been the recipient of Defra funding for HPAI responsive analysis.
Dan Haydon
None raised
Lonneke Vervelde
Recipient of UKRI and Defra funding under the “FluMap” project led by Dr. Ian Brown at APHA.
Sarah Burthe
None raised
James Pearce-Higgins
Oversees the JNCC (Joint Nature Conservation Committee) and BTO (British Trust for Ornithology) Partnership that much of the BTO’s term biodiversity surveillance and this year has included additional work on HPAI. Multiple government funded contracts, including from Defra, support various areas of BTO research.
Rob Robinson
Oversees the JNCC and BTO Partnership that much of the BTO’s term biodiversity surveillance and this year has included additional work on HPAI. Multiple government funded contracts, including from Defra, support various areas of BTO research.’
Kath Webster
None raised
Munir Iqbal
Recipient of UKRI and Defra funding under the “FluMap” project led by Dr. Ian Brown at APHA.
Nigel Gibbens
None raised
Stephen Hinchliffe
None raised
Annex B – Glossary
ADS Avian demographic schemes
AON Apparently occupied nest
AOT Apparently occupied territories
APHA Animal and Plant Health Agency
AIV Avian influenza virus
BTO British Trust for Ornithology
CNCB Country nature conservation bodies
CSA Chief Scientific Adviser
CVO Chief Veterinary Officer
Defra Department for Environment, Food and Rural Affairs
DIVA Differentiating infected from vaccinated animal
FMD Foot and mouth disease
FSA Food Standards Agency
GBPR Great Britain poultry register
GSMP Goose and swan monitoring programme
HA Haemagglutinin
HPAI Highly pathogenic avian influenza
HPAIG Highly pathogenic avian influenza Group
HPAIV Highly pathogenic avian influenza Virus
IPCC Intergovernmental Panel of Climate Change
IPs Infected premises
JNCC Joint Nature Conservation Committee
LPAI Low pathogenicity avian influenza
MDA Maternally derived antibodies
NE Natural England
NEEG National Emergency Epidemiology Group
PCR Polymerase chain reaction
RSPB Royal Society for Protection of Birds
SAC Science Advisory Council
SAC-ED Science Advisory Council Exotic and Emerging Animal Diseases
SMP Seabird monitoring programme